Chlorofluorocarbons Background
Excerpt from Environmental Tracers in
Subsurface Hydrology
Peter Cook and Andrew Herczeg(eds.) Kluwer Acadmic Press
In This Document
- Introduction
- History of Atmospheric CFC Mixing Ratios
- Enrichment of Air Mixing Ratios
- Unsaturated Zone Transport of CFCs
- Principles of Chlorofluorocarbon Dating
- Dating with other Atmospheric Gases
- Processes that can Modify Apparent CFC Age
- Field Examples
- Concluding Remarks
- References
Introduction
Chlorofluorocarbons(CFCs) are stable, synthetic, halogenated alkanes, developed in the early 1930s as safe alternatives to ammonia and sulphur dioxide in refrigeration. Production of CFC-12 (dichlorodifluoromethane, CF2Cl2) began in 1931 followed by CFC-11 (trichlorofluoromethane) in 1936. Many other CFC compounds have since been produced, most notably CFC-113 (trichlorotrifluoroethane, C2F3Cl3). CFCs are nonflammable, noncorrosive, nonexplosive, very low in toxicity, and have physical properties conducive to a wide range of industrial and refrigerant applications. Primary uses of CFC-11 and CFC-12 include refrigerants in air-conditioning and other coolers, blowing agents in foams, insulation, and packing materials, propellants in aerosol cans, and as solvents. CFC-113 has been used primarily by the electronics industry in manufacture of semiconductor chips, in vapour degreasing and cold immersion cleaning of microelectronic components, and as a solvent in surface cleaning procedures (Jackson et al., 1992). Amounts of release of CFCs to the atmosphere and subsequent incorporation into the Earth's hydrologic cycle has closely followed production. For example, it has been estimated that CFC-11 and CFC-12 produced for aerosol propellants were released, on average, within 6 months of sale (Gamlen et al., 1986), and emissions of CFCs used as blowing agents in open-cell foams and extruded foams took place within less than 1 year of manufacture of the chemicals (Midgley and Fisher, 1993). CFCs used in refrigeration and airconditioning have somewhat greater storage times, being released on average within 10 years, and CFCs used as blowing agents in closed-cell thermoset foams are released after more than 10 years (Midgley and Fisher, 1993). Current estimates of the atmospheric lifetimes of CFC-11, CFC-12, and CFC-113 are 45± 7, 87± 17, and 100± 32 years (Volk et al., 1997).
CFCs provide excellent tracers and dating tools of young water (1940 to present time scale). CFC-11, CFC-12 and CFC-113 concentrations in water can be determined to a detection limit of about 0.3 picograms per kg of water (pg/kg-1) using purge and trap, gas chromatographic techniques with electron-capture detector (GC-ECD; Bullister, 1984; Bullister and Weiss, 1988; Busenberg and Plummer, 1992). By measuring concentrations of CFC-12, CFC-11 and CFC-113, it is possible to identify groundwater recharged since approximately 1941, 1947, and 1955, respectively. Groundwater dating with CFC-11, CFC-12 and CFC-113 is possible because (1) the atmospheric mixing ratios of these compounds are known and/or have been reconstructed from 1940 to the present, (2) the Henry's law solubilities in water are known, and (3) concentrations in air and young water are relatively high and can be measured.
The feasibility of using CFCs as tracers of recent recharge and indicators of groundwater age was first recognized in the 1970s (Thompson et al., 1974; Schultz et al., 1976; Randall and Schultz, 1976; Thompson, 1976; Hayes and Thompson, 1977; Randall et al., 1977; Thompson and Hayes, 1979; Schultz, 1979). Thompson et al. (1974) conducted tracer tests by injecting fluorescein dye, and later a solution containing 100 mg kg-1 of CFC-11 into an aquifer of poorly sorted sand and gravel. The fluorescein dye was not recovered whereas the CFC-11 arrived at a nearby monitoring well within the expected travel time. This indicated the potential for use of CFCs as tracers in porous media where moderately adsorbing tracers could not be used. Schultz et al. (1976) demonstrated the use of CFC-11 in tracing groundwater recharge of sewage effluent discharged to dry washes and riverbeds near Tucson, Arizona. Randall and Schultz (1976) introduced a method to date a water sample with CFC-11 in relation to an analysis of another water sample of known age, and to determine age from analysis of a single water sample through Henry's law solubility. Randall and Schultz (1976) also recognised the need for reconstruction of atmospheric mixing ratios of CFCs to improve dating capability, the possibility of detecting both CFC-11 and CFC-12 in the same analysis (at that time, analytical procedures were capable of detecting only CFC-11), and potential for dating using ratios of CFCs. Thompson (1976), Hayes and Thompson (1977), and Thompson and Hayes (1979) refined field GC-ECD analytical procedures for CFC-11 and tested the feasibility of dating with CFC-11 in groundwater systems in parts of New Jersey, Arkansas, and Texas, where hydrologic conditions were well established. In all three cases they found good agreement between CFC-11 concentrations in the groundwater and known hydrologic conditions.
Since the late 1970s, CFCs have been used increasingly as tracers of oceanic circulation, ventilation, and mixing processes (Bullister, 1989; Rhein, 1991; Smethie, 1993; Wallace et al., 1994; Warner et al., 1996; Bönisch et al., 1997, and references therein). The Henry's law solubilities of CFC-11, CFC-12 and CFC-113 were measured to high precision (Warner and Weiss, 1985; Bu and Warner, 1995), and purge and trap GC-ECD analytical procedures improved (Bullister and Weiss, 1988). Much of this work developed by the oceanographic scientific community was adopted for groundwater studies in the early 1990s (Epler, 1990; Busenberg and Plummer, 1991, 1992).
Methods of collection and preservation of water samples prior to analysis have also evolved. In the first groundwater studies (1970s), CFC analyses were performed in the field immediately after collection in glass syringes. Because of Nuclear Regulatory Commission (NRC) licensing requirements for the radioactive 63Ni source in the ECD, it is no longer practical to measure CFC concentrations on site for most groundwater investigations. For this reason, Busenberg and Plummer (1992) designed sampling equipment and introduced procedures for collection and preservation of water samples in the field. The samples are then transported to the NRC-licensed laboratory for analysis. In field sampling, a closed path is established between the well or pump through refrigeration-grade copper tubing in order to flush and cap a 125ml glass bottle with an aluminum foil lined cap. The sample collection procedure is conducted under water in a beaker to isolate the atmosphere from the water sample in the bottle.
In the following, we discuss the interpretation of temporal aspects of CFC concentrations measured in air, soil air, and groundwater. Applications of CFC data to hydrologic studies are discussed, based on a series of groundwater studies (Busenberg and Plummer, 1992; Dunkle et al, 1993; Busenberg et al., 1993; Plummer et al., 1993; Ekwurzel et al., 1994; Reilly et al., 1994; Katz et al., 1995; Cook et al., 1995, 1996; Cook and Solomon, 1995, 1997; Szabo et al., 1996; Oster et al., 1996; Johnston et al., 1998; Plummer et al., 1998 a,b).
History of Atmospheric CFC Mixing Ratios
Production and release of CFCs to the atmosphere rose rapidly through the 1970s and 1980s (AFEAS, 1997). Annual production of CFC-11 and CFC-12 peaked in 1987 and that of CFC-113 peaked in 1989. Total production of CFC-11 and CFC-12 in 1987 was 7.0 and 9.4 million metric tons, respectively, and that of CFC-113 in 1989 was 1.7 million metric tons. Although CFCs are nontoxic, nonflammable and noncarcinogenic, CFCs are a prime contributor to stratospheric ozone depletion. In 1987, through signing of the Montreal Protocol on Substances That Deplete the Ozone Layer, 37 nations agreed to limit release of CFCs and to halve CFC emissions by the year 2000. The protocol was strengthened in 1990, requiring industrial countries to phase out CFCs completely by the year 2000, and developing countries to do so by 2010. Further strengthening of the protocol occurred in 1992 with 90 countries agreeing to cut off production of CFCs by 1996. Production of CFCs ceased in the USA as of January 1, 1996. Through 1995, total worldwide production of CFC-11 exceeded 8.6 million metric tons, that of CFC-12 was nearly 11.3 million metric tons, and that of CFC-113 was nearly 2.7 million metric tons. AFEAS (1997) estimated that, through 1995, 87.2% of the CFC-11, 95% of the CFC-12, and 99% of the CFC-113 cumulative production had been released. Most of the unreleased CFC-11 resides in closed-cell foam products, and most of the unreleased CFC-12 is in non-hermetically-sealed refrigeration.
Air mixing ratios of CFC-11 and CFC-113 peaked in about 1993 and 1994, respectively, in North America with values of 275.1 and 85.4 parts per trillion, volume (pptv), respectively (Elkins et al., 1993). The global average CFC-11 tropospheric mixing ratio peaked at 272 pptv in 1993 and has since declined slowly (Elkins et al., 1993, 1996). The CFC-12 mixing ratio continued to rise through 1998, but the rate of increase in the CFC-12 mixing ratio slowed to a rate of about 1.66 pptv each year from 1988 to 1998. CFC-12 concentration peaked with a global average tropospheric mixing ratio of 544 pptv in mid-1999. In 1995, air in North America (based on measurements from Niwot Ridge, Colorado, an atmospheric monitoring station in the Rocky Mountains at 3,013 m elevation near Boulder, Colorado) contained about 274 pptv of CFC-11, 534 pptv of CFC-12 and 85 pptv of CFC-113 (Elkins et. al., 1996). Figure 1 shows air mixing ratios in pptv for CFC-11, CFC-12 and CFC-113 based on National Oceanic and Atmospheric Administration (NOAA) measurements from
Atmospheric Input Functions for GW Dating
Niwot Ridge, Colorado (1976 to present). CFC-11 and CFC-12 mixing ratios for air before 1976 were reconstructed from production data by McCarthy et al. (1977). Air mixing ratios of CFC-113 were compiled from Wisegarver and Gammon (1988), Tominaga (1992), Fisher and Midgley (1993), Fraser et al. (1996), Busenberg and Plummer (1993; unpublished data, 1998), and data from NOAA (http://www.cmdl.noaa.gov).
As most CFCs have been released to the troposphere in the northern hemisphere, CFC mixing ratios in the southern hemisphere lag slightly behind those of the northern hemisphere. For example, in 1990, air sampled at Tuluila, American Samoa (14.23°S 170.56°W) and Cape Grim, Tasmania, Australia (40.41°S 144.64°E) had about 20 pptv less CFC-12 than air at Niwot Ridge, Colorado (40.04°N 105.54°W), and at these same sites, CFC-11 was about 14 pptv less than that measured at Niwot Ridge (Elkins et al., 1996). For CFC dating in other parts of the world, especially the southern hemisphere, the historical mixing ratios can be reconstructed. The Climate Monitoring and Diagnostics Laboratory (CMDL) of the NOAA, routinely measures CFC concentrations at stations throughout the world. Long-term monitoring of CFCs from the ALE/GAGE/AGAGE network (Cunnold et al., 1997) is available for Adrigole/Mace Head, Ireland; Cape Meares, Oregon (until 1989); Ragged Point, Barbados; Cape Matatula, Samoa; and Cape Grim, Tasmania through the Carbon Dioxide Information and Analysis Center, Oak Ridge, TN (http://cdiac.esd.ornl.gov/ndps/alegage.html).
Enrichment of Air Mixing Ratios
Near urban areas, air mixing ratios may be enriched in CFCs relative to background air composition. Air sampled from a 496 m tower in North Carolina, southeast USA (Hurst et al., 1997) over a 15-month period from November, 1994 through January, 1996 closely tracked the Niwot Ridge CFC-11 background mixing ratios with relative standard deviations of only 0.8%. Over the same period, CFC-12 mixing ratios averaged about 10 pptv higher than the Niwot Ridge background values. Minimum CFC-12 values from the North Carolina site were at or very near the Niwot Ridge background values, but overlain frequently with spikes of CFC-12 that were more than 50 pptv above the Niwot Ridge values. The relative standard deviations of the CFC-12 mixing ratios from North Carolina were 1.7%, twice that of CFC-11. The CFC-12 anomalies were attributed to local and regional sources.
The highest emissions of CFCs occur in the eastern United States and western Europe (McCulloch et al., 1994). Oster et al. (1996) described a series of 8000 CFC air measurements from four sites in western Europe over the period 1989 to 1992. CFC excesses relative to global background air averaged 125% (CFC-11) and 62% (CFC-12) at an urban site (Heidelberg, Germany) and 34% (CFC-11) and 24% (CFC-12) at a rural site (Wachenheim, Germany). These authors detected regular diurnal, weekly and annual cycles in the CFC mixing ratios, as well as episodic variations attributed to local emissions and changing air masses.
Ho et al. (1998) monitored CFC-11 and CFC-12 atmospheric mixing ratios over a 16-month period (July, 1996-October, 1997) at a site 25 km north of New York City, NY. Atmospheric mixing ratios of CFC-11 and CFC-12, analyzed at approximately 10 minute intervals averaged 6 and 13% higher than remote northern hemisphere atmospheric mixing ratios. Ho et al. (1998) found diurnal, weekly and seasonal cycles in CFC atmospheric mixing ratios. CFC excesses were lower in the winter months than during the summer and attributed to both seasonal emission variations and atmospheric transport patterns. Wang et al. (1998) collected 51 air samples randomly over a 1-hour period throughout the Taipei metropolitan area in January, 1997. The concentrations of CFC-11 and CFC-113 were found to be uniform and close to background values. In contrast, CFC-12 concentrations were typically more than 100 pptv higher than background values and exhibited area dependence.
Unsaturated Zone Transport of CFCs
CFCs provide nearly ideal tracers for investigating unsaturated zone gas transport processes. Physical processes affecting gas transport in the unsaturated zone include diffusion, and pressure flow in response to changes in barometric pressure or thermal gradients. Air transport driven by barometric pumping is thought to reach only a few metres' depth in homogeneous, porous unsaturated zones (Massmann and Farrier, 1992; Severinghaus et al., 1997). Horizontal pressure gradients caused by variations in land surface relief can cause air movement of meters or tens of meters into the unsaturated zone during storm events (Massmann and Farrier, 1992). Beyond a few meters, diffusive processes play an increasing role in determining deep unsaturated zone gas composition in homogeneous, porous soils.
At four field sites in the Southern High Plains of Texas, Weeks et al. (1982) found detectable concentrations of CFC-11 and CFC-12 to depths of 43.9 m. CFC-12 concentrations were only about 80% of modern air at 10 m, and fell with depth to about 10% of air mixing ratios at 43.9 m. Weeks et al. (1982) showed that the vertical CFC profiles could be modeled by accounting for effects of soil porosity, tortuosity, gas-liquid partitioning, sorption and diffusion. In undisturbed unsaturated zones, where groundwater recharge was small, diffusion was the primary mechanism for downward migration of CFCs. Numerical modelling of the CFC profiles permitted determination of tortuosity values that were in relatively good agreement with tortuosity values determined from theoretical and empirical relationships.
Busenberg et al. (1993) measured CFC profiles in unsaturated zone playa sediments and in the underlying Snake River Plain basalts to depths of nearly 60 m in southern Idaho. The piezometer nests were first sampled for CFCs in 1979 and again in 1991. In 1991, CFC-11 and CFC-12 concentrations at 57.5 m were 7% and 27% of the respective soil air concentrations measured at 3 m. Although both CFC mixing ratios fell dramatically along the vertical profile, CFC-11 was depleted relative to CFC-12, as predicted by the soil-gas diffusion model (Weeks et al., 1982).
To evaluate the feasibility of using sand dunes as an archive of old air, Severinghaus et al. (1997) measured profiles of CFC-11, CFC-12, CFC-113, 85Kr, CO2, N2O, CH4 and O2 to depths of 60 m in two test holes in the Algodones Dunes, Imperial Valley, California. Elevated concentrations of CO2 and N2O and depressed concentrations of CH4 and O2 were evidence of microbial processes within the dunes. However, the CFC profiles closely matched results from a pure diffusion model for CFC-11, CFC-12 and CFC-113. The few 85Kr measurements were also consistent with pure diffusion.
In fractured rock, barometric pumping can transport gas through hundreds of metres on time scales of months, rather than the few metres and decades required for diffusive transport (Nilson et al., 1991). Studies of gas flow in boreholes in Yucca Mountain, Nevada indicate a combination of thermal-topographic, barometric, and wind effects controlling unsaturated zone CFC advection on time scales of ≤5 years (Thorstenson et al., 1998).
Knowledge of the concentrations of CFCs in the unsaturated zone is important to interpretation of CFC concentrations in groundwater. CFC dating of groundwater is based on the assumption that historical CFC concentrations in the unsaturated zone, or in the air that was last in contact with the water, are known or can be calculated. The effect of unsaturated zone thickness on CFC dating of groundwater is discussed in the section on unsaturated zone thickness
Principles of Chlorofluorocarbon Dating
Setting the ‘Clock'
Groundwater age, when based on measurement of the concentration of man made tracer gases in water, refers to the time elapsed since recharge and isolation of the newly recharged water from the soil atmosphere. The rate at which a parcel of water becomes isolated from the unsaturated zone air is, in part, a function of recharge rate, porosity of the unsaturated zone soil, aqueous and gaseous diffusion coefficients, and magnitude of water table fluctuations. Schlosser et al. (1989) discussed theoretical aspects of gas confinement as it relates to helium in 3H/3He dating. They found that significant loss of 3He occurs when the vertical velocity of water infiltration through the unsaturated zone is less than about 0.25-0.5 m yr-1. Through detailed sampling of vertical profiles at a field site near Sturgeon Falls, Ontario, Solomon et al. (1993) found that shallow groundwater lost 3He when the water table fell from near land surface to 2 m depth. Therefore, the 3H/3He clock was set at the seasonal low in the water table. Cook et al. (1995) measured CFC concentrations in air and in shallow groundwater at the Sturgeon Falls site. The shallow groundwater sampled near the seasonal water table low of 1.5 m retained the CFC concentrations formed when the water table was near land surface and in equilibrium with the atmosphere. As pointed out by Cook and Solomon (1997), CFCs have higher solubility and lower diffusion coefficients than 3He. Thus, once water is recharged at the water table, there is less gas exchange with CFCs than with helium. So, at least at the Sturgeon Falls site, the CFC clock appears to be set at recharge (season high in the water table), whereas the 3H/3He clock is set at the seasonal low in the water table. For most dating applications, CFC confinement is thought to occur fairly rapidly; some time between the seasonal high and low water table (Cook and Solomon, 1997). Shallow groundwater remains closed to gas exchange because molecular diffusion coefficients of gases are some 5 orders of magnitude smaller in water than in air.
Groundwater age, model age, apparent age
Although reference is often made to dating of groundwater, the age actually applies to the date of introduction of the chemical substance, and not the water. Unless we recognise and account for all the physical and chemical processes that affect the concentrations of CFCs in the aquifer, the CFC-based age is not necessarily equal to the transit time of the water. The accuracy of the determined age depends in part on how perfectly the CFCs are transported with the aqueous phase. The concentrations of all dissolved substances are affected, to some extent, by transport processes. For CFCs, the concentrations can also be affected by chemical processes, such as degradation and sorption during transit. For this reason, the term ‘age' is usually qualified with the word ‘model' or ‘apparent', that is, ‘model age' or ‘apparent age'. The emphasis on model or apparent ages is needed because simplifying assumptions regarding the transport processes have been made in assigning the CFC-based apparent, or model age, and other chemical processes that may be affecting the CFC concentration in water are usually not accounted for.
The simplest and most common transport assumption in CFC-based dating is to assume piston flow. The interpreted age assumes that the concentration of the CFC was not altered by transport processes from the point of entry to the measurement point in the aquifer. Most of the apparent CFC ages referred to in the literature and in this chapter are based on the simplifying assumption of piston flow. While the term ‘apparent age' refers to the duration since recharge and isolation from the atmosphere, we will also refer to ‘recharge date', which is the date of recharge implied by the measured concentration of the CFC compound in the water. It is important to use recharge date, especially when interpreting temporal significance from the concentrations of other solutes in groundwater (e.g. nitrates) that may have transient input behaviour.
Dating groundwater with CFCs
Groundwater dating with CFCs is based on Henry's
law solubility. That is, the concentration of the gas dissolved
in water in equilibrium with air is proportional to the partial
pressure, pi, of the gas in air. The term pi
is defined (Warner and Weiss, 1985)
where xi is the dry air mole fraction
of the ith CFC (xi < < 1), P
is the total atmospheric pressure and pH2O is the
water vapor pressure. For ideal gases, the dry air mole fraction
is replaced with the air mixing ratio, volume per volume.
Henry's law then gives the CFC solubility in water, Ci
where KH is the Henry's law constant
for the ith CFC. KH has been measured
in pure water and seawater for CFC-11 and CFC-12 (Warner and
Weiss, 1985), and for CFC-113 (Bu and Warner, 1995). Table
1 gives least squares fitting parameters to the temperature
(Kelvin) and salinity (parts per thousand, ‰) dependence of
KH for concentrations in mol kg-1 atm-1
and mol L-1 atm-1, valid for temperatures
of 273-313 Kelvin (0-40 °C), and salinities of
0-40‰ in the equation
Table 1 Constants for calculation of KH. (Warner and Weiss, 1985; Bu and Warner,1995)
CFC |
a1 |
a2 |
a3 |
b1 |
b2 |
b3 |
Solubilities in mol kg-1 atm-1 |
||||||
CFC-11 |
-136.2685 |
206.1150 |
57.2805 |
-0.148598 |
0.095114 |
-0.0163396 |
CFC-12 |
-124.4395 |
185.4299 |
51.6383 |
-0.149779 |
0.094668 |
-0.0160043 |
CFC-113 |
-136.129 |
206.475 |
55.8957 |
-0.02754 |
0.006033 |
--- |
Solubilities in mol L-1 atm-1 |
||||||
CFC-11 |
-134.1536 |
203.2156 |
56.2320 |
-0.144449 |
0.092952 |
-0.0159977 |
CFC-12 |
-122.3246 |
182.5306 |
50.5898 |
-0.145633 |
0.092509 |
-0.0156627 |
CFC-113 |
-134.243 |
203.898 |
54.9583 |
-0.02632 |
0.005874 |
--- |
In assigning an apparent age to a CFC concentration
measured in a water sample, the measured concentration is
converted to units of mol kg-1 (or mol L-1)
and then to a dry air atmospheric mixing ratio, xi.
In this process the Henry's law constant must be calculated
at the recharge temperature, that is, the temperature at the
water table during recharge (see discussion below). The Henry's
law constant is also a function of salinity. But, as a general
rule, most shallow groundwater of interest in dating with
CFCs is too dilute to require corrections for salinity. For
example, if salinity corrections were ignored, a 1 year (too
old) bias in apparent CFC age results for the following salinities
and recharge dates: 10‰ for the 1960s, 5‰ for the 1970s, 2‰
for the 1980s, and 1‰ for the early 1990s. Salinity corrections
will be necessary for all studies involving seawater, estuaries,
and saline lakes. The recharge elevation is also needed to
estimate the total atmospheric pressure, P, during recharge.
For recharge elevations less than 3000 m, the relation,
ln P = - H/8300 (List, 1949), can be used,
where H is the recharge elevation in metres. Because recharge
occurs in a moist unsaturated zone, relative humidity is near
100%. Therefore, to solve for the dry air mixing ratio of
the particular CFC, the vapor pressure of water, pH2O,
is subtracted from the total pressure. The vapor pressure
of water is (Weiss and Price, 1980)
The dry air mixing ratio for the particular CFC, xi, is then compared to the appropriate historical atmospheric mixing ratio (Figure 1) to determine recharge date. Apparent age is simply the date of sampling minus the recharge date.
As we can use Henry's law to interpret apparent CFC ages for individual CFC compounds, there are also variations in the ratios of atmospheric CFC concentrations that can be used for dating. Figure 2 shows reconstruction of the ratios of CFC-11/CFC-12 and CFC-113/CFC-12 in remote North American air. The ratio of CFC-11/CFC-12 in air increased dramatically from the late 1940s to about 1977, and that of CFC-113/CFC-12 had a modest increase during the 1980s.
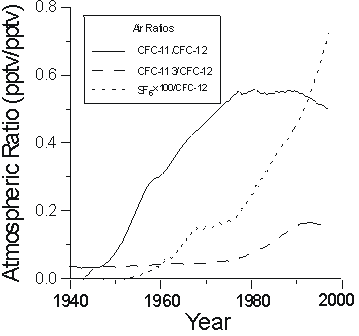
Figure 2 Atmospheric ratios of CFC-11/CFC-12, CFC-113/CFC-12, and SF6/CFC-12.
Dating with other Atmospheric Gases, Sulfurhexafluoride
The slowing and turnover of CFC atmospheric mixing ratios in the 1990s has resulted in ambiguity in CFC-based apparent age. Sulphur hexafluoride (SF6) has been developed as an alternative to CFCs for gas-solubility dating of water (Busenberg and Plummer, 1997). Significant production of SF6 began in the 1960s for use in high voltage electrical switches. SF6 is extremely stable, with an estimated atmospheric lifetime of 800 (Morris et al., 1995) to 3200 years (Ravishankara et al., 1993). SF6 can be analysed by GC-ECD techniques to a precision of 1-3% (Wanninkhof et al., 1991; Law et al., 1994), so, even though atmospheric mixing ratios are small (currently about 10 pptv), dating is possible from about 1970 to the present.
The earliest reported measurement of the atmospheric concentration of SF6 was 0.03 pptv in 1970 (Lovelock, 1971). SF6 is accumulating rapidly in the atmosphere with a current growth rate of more than 7% per year. The historical atmospheric mixing ratio of SF6 has been reconstructed from production records and atmospheric measurements (Ko et al., 1993; Elkins et al., 1996; Maiss et al., 1996; Geller et al., 1997; Levin and Hesshaimer, 1996), and retrieved from concentrations measured in seawater (Law et al., 1994) and in previously-dated groundwater (Busenberg and Plummer, unpub. data). Figure 1 shows a preliminary reconstruction of northern hemisphere SF6 mixing ratios. As atmospheric CFC concentrations fall, an even more sensitive dating tool will be the ratio of SF6 to, for example, CFC-12 (Figure 2). However, because of the low solubility of SF6 (Mroczek, 1997), apparent ages can be very sensitive to excess air, so this needs to be accurately determined.
Busenberg and Plummer (1997) used SF6 successfully to date shallow groundwater on the Delmarva Peninsula, Maryland, and water from springs in the Blue Ridge Mountains of Virginia. Although SF6 is mostly of anthropogenic origin, concentrations between one and two orders of magnitude higher than that possible for modern air-water equilibrium were measured in groundwater from volcanic rift zones in New Mexico and Idaho (Busenberg and Plummer, 1997). There is likely a natural, igneous source of SF6 that will complicate dating in some environments. SF6 has also been used as an artificial tracer (Wilson and Mackay, 1996; Schlatter et al., 1997) that is injected into groundwater or surface water. Clark et al. (1995b) found that wastewater discharged to the Hudson River at New York City was contaminated with SF6. Busenberg and Plummer (1997) found minor contamination of SF6 in Rio Grande water near an outfall from a sewage treatment plant. All other surface waters had SF6 concentrations near equilibrium with air.
Processes that can Modify Apparent CFC Age
Many processes occur during recharge and in the groundwater environment that can affect CFC concentrations beyond those set by air-water equilibrium, and consequently affect interpretation of apparent age. Table 2 lists the most important processes, their effect on apparent CFC age, and relative importance.
Table 2 Summary of processes that can modify apparent age.
Property |
Environment Most Affected |
Description of Process |
Effect on Apparent Age |
Recharge temperature |
Shallow water table |
Temperature at the water table during recharge. |
|
Over-estimated...................... | Too young | ||
Under-estimated.................... | Too old | ||
± 2°C, ≤1970 ± 1 year or less ± 2°C, 1970-1990, ± 1-3 years ± 2°C, >1990, >3 years |
|||
Excess air |
Rapid, focused recharge; fractured rock. |
Addition of air trapped and dissolved during recharge. Significant for post-1990 recharge. |
Too young |
Recharge elevation |
Mountain recharge |
Water recharged at high altitude dissolves less CFCs because of lower barometric pressure. |
|
Over-estimated...................... | Too young | ||
Under-estimated.................... | Too old | ||
± 100 m not important ± 1000 m, < 1987, ± few years Significant for post-1990 recharge |
|||
Thickness of unsaturated zone |
Unsaturated zone >10 m |
Air in deep unsaturated zone is older than that of the modern troposphere. 0-10 m, error < 2 years 30 m, error 8 - 12 years. |
Too old |
Urban air |
Eastern USA, Western Europe, urban areas |
CFC mixing ratios in urban and industrialised areas can exceed regional values. |
Too young |
CFC contamination |
Urban and industrial areas, sewage effluent |
CFCs added to water from local anthropogenic sources, in addition to that of air-water equilibrium. |
Too young (impossibly young) |
Microbial degradation |
Anaerobic environments, sulphate-reducing, methanogenic Fluvial and glacial drift sediment |
No degradation in aerobic environments........ |
No effect |
Sulphate-reducing, and fermentation: CFC-11, CFC-113 degraded, CFC-12 quasi-stable....... | CFC-11, CFC-113 Too old | ||
Methanogenic: CFC-11 ≥ CFC-113>>CFC-12 | Too old | ||
Sorption |
Organic-rich sediment, peat |
Sorption of CFCs onto particulate organic carbon and mineral surfaces. CFC-113>>CFC-11≥CFC-12 |
Too old |
Mixed waters |
Production wells, fractured rock |
Mixing of young and older water in water pumped from open intervals in wells |
|
Apparent age of young fraction in mixture..... | Too old | ||
Apparent age of old fraction in mixture......... | Too young | ||
Hydrodynamic dispersion |
All groundwater environments |
Generally small effect for CFCs. |
|
1975 - 1993..................... | Too old | ||
<1975............................... | Too young |
Recharge temperature, pressure, and excess air
The recharge temperature is the temperature at the water table during recharge. Figure 3 shows concentrations of CFC-11, CFC-12, and CFC-113 in pg kg-1 expected in groundwater recharged between 1940 and 1998, at sea level, and in equilibrium with remote North American air (Figure 1) for recharge temperatures of 5 to 25 °C. The plots show the importance of recharge temperature in interpreting apparent age of groundwater with CFCs. For example, CFC-11 concentrations in water recharged in 1990 at 5°C are more than double the CFC-11 concentrations if recharged at 25 °C. Figure 3 can be used to interpret the
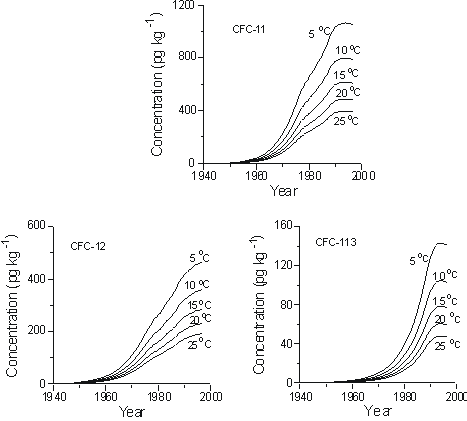
Figure 3 Concentrations of CFC-11, CFC-12, and CFC-113 in water recharged between 1940 and 1998 at temperatures of 5 to 25 °C at sea level.
magnitude of age errors that result from uncertainties in recharge temperature. For example, if the CFC-11 concentration was 600 pg kg-1 in a particular water sample that recharged at 15°C, but recharge temperature was erroneously assumed to be 5°C, an apparent age that is some 12 years too old would be assigned, based on CFC-11. An uncertainty in recharge temperature of ± 2°C results in age uncertainties of 3 years or less for water recharged prior to about 1990 (Busenberg et al., 1993). Over-estimation of recharge temperature results in apparent ages too young, and under-estimation gives ages that are biased old. Uncertainty in recharge temperature of ± 2°C leads to uncertainty in apparent CFC ages of 1 year or less for water recharged prior to the mid-1970s. However, as the air mixing ratios peaked in the mid- and late-1990s, CFC apparent ages became extremely sensitive to uncertainties in recharge temperature.
Gas solubilities are also a function of total pressure. Average barometric pressure during recharge is not normally known, but can be estimated from the recharge elevation (as discussed above). If the pressure is overestimated, the interpreted CFC age will be biased old. Uncertainty in atmospheric pressure does not normally introduce significant errors in CFC dating in areas of low relief where elevation of recharge is often known within a few hundred meters. Uncertainty in recharge elevation of 1000 m results in age uncertainty of a few years or less for waters recharged prior to 1987 (Busenberg et al., 1993). However, in mountainous terrain, uncertainty in recharge elevation can be large and have a more significant effect on interpreted apparent age.
Excess air is air dissolved in groundwater in relative proportions equal to that of the troposphere, and in excess of that determined by solubility equilibrium. Excess air is trapped and dissolved under increased hydrostatic pressure in groundwater at the capillary fringe or in fractures as the water table rises. Wilson and McNeill (1997) compiled most of the published values of recharge temperature and excess air determined from noble gas measurements of groundwater. Excess air content varies with hydrogeologic environment, but is usually less than 6 cm3 L-1. Estimates of recharge temperature and excess air can be determined from noble gas measurements, or, in the absense of denitrification, from measurements of dissolved nitrogen and argon.
Introduction of excess air adds CFCs to groundwater, and if not accounted for in age interpretation, causes a young-age bias. However, the effect is small and can be ignored in most groundwater cases (Busenberg and Plummer, 1992), the exception being dating of post-1990 groundwater. Because atmospheric mixing ratios of CFCs are leved off, dating becomes extremely sensitive to introduction of even small amounts of CFCs from excess air (and/or uncertainty in recharge temperature).
Unsaturated zone thickness
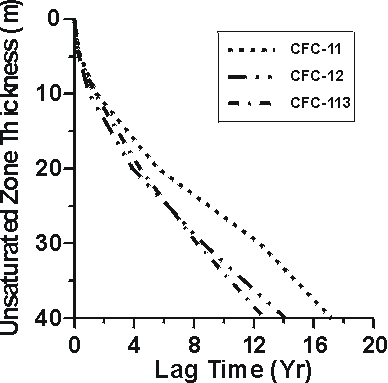
Figure 4 Lag times for water recharged through CFC-diffusion profiles in unsaturated zone air of 0-40 metres thickness (Cook and Solomon, 1995).
The apparent CFC age is based on the assumption of equilibrium of infiltration water with tropospheric air at the time of recharge. With increasing thickness of the unsaturated zone, unsaturated zone gas transport processes play a larger role in the interpretation of apparent CFC age.
The CFC profiles measured and modeled in unsaturated zone playa sediments and in the underlying Snake River Plain basalts to depths of nearly 60 m in southern Idaho (Busenberg et al., 1993) can be used to investigate groundwater recharge through deep unsaturated zones. If soil water equilibrated with the soil air at the water table at the time of unsaturated zone gas sampling (1991), shallow groundwater in the Snake River Plain aquifer would have apparent recharge dates of 1968 and 1971, based on CFC-11 and CFC-12, respectively. Thus, water recharged at this site in 1991 would have apparent CFC-11 and CFC-12 ages that would be biased old (lagged) by 23 and 20 years, respectively. Cook and Solomon (1995) presented theoretical calculations of the lag times for apparent CFC-11, CFC-12 and CFC-113 ages for water recharged through thick unsaturated zones. They found lag times were mostly dependent on gas solubility, gas diffusion coefficient, and soil water content. Figure 4 shows CFC-11, CFC-12, and CFC-113 lag times from Cook and Solomon (1995) calculated for water recharged through unsaturated zones of 0-40 m with unsaturated zone volumetric water content of 0.15. The results of Cook and Solomon (1995) suggest that for shallow unsaturated zones (<10 m), lag times will be less than 2 years, and for most purposes can be ignored. Johnston et al. (1998) found CFC-11 apparent ages of groundwater beneath a 25m thick unsaturated zone lagged 6-10 years behind 3H/3He ages, consistent with the diffusion mechanism.
The extent to which unsaturated gas transport processes affect the apparent CFC age depends also on the predominant recharge mechanism and physical, hydraulic properties of the unsaturated zone. In arid and semi-arid environments, recharge can occur as continuous, diffuse infiltration of water, maintaining close contact with the unsaturated zone air, or as transient, focused recharge in response to, for example, flood events in arroyos, that bypasses most water contact with the soil air (Gee and Hillel, 1988). Depending on recharge mechanism, water may fully equilibrate with the unsaturated zone air during recharge, or retain CFC concentrations acquired at the land surface prior to recharge.
CFC concentrations in groundwater in southern Idaho beneath thick unsaturated zones show cases of both focused recharge, and diffuse infiltration (Busenberg et al., 1993; Busenberg and Plummer, 1995, unpub. data). Water apparently recharged by focused recharge is relatively young, exhibits little or no separation in CFC-11 and CFC-12 apparent ages, and CFC apparent ages tend to agree with those based on 3H/3He. Water recharged by diffuse infiltration is older in apparent CFC age relative to apparent ages based on 3H/3He.
Urban air
Szabo et al. (1996) reported apparent contamination of CFC-11 and CFC-12 concentrations in shallow groundwater from rural research sites in southern New Jersey located downwind of two large metropolitan areas: Philadelphia, Pennsylvania, and Wilmington, Delaware. CFC-11 and CFC-12 concentrations in shallow groundwater at the southern New Jersey sites were higher than could be possible for equilibrium with North American air. Air was not sampled at the New Jersey site, but air from the Delmarva Peninsula, about 60 km south of the New Jersey site, was about 4% higher in CFC-11 and CFC-12 than corresponding values measured at Niwot Ridge, Colorado (Szabo et al., 1996).
CFC profiles in the unsaturated zone from parts of western Europe show that local air anomalies in CFC emissions are damped by diffusive mixing processes in the unsaturated zone (Oster et al., 1996), and therefore it may be possible to date shallow groundwater in some urban areas, if local, historic unsaturated zone mixing ratios can be reconstructed. In some areas, groundwater may provide an archive of local CFC mixing ratios that may be dated by other techniques and used for other local studies.
Ho et al. (1998) discuss implications of urban air on groundwater dating with CFCs. For example, water recharged in urban areas prior to 1987 in equilibrium with air having CFC-11 and CFC-12 excesses that are 10% higher than North American air will be biased young by 2 years or less. However, the errors resulting from urban air become very large in the 1990s, due to the levelling off of the CFC atmospheric mixing ratios.
Groundwater contamination
For the purposes of CFC dating, we use the term ‘contaminated' to refer to water that has CFC concentrations that are greater than that possible for equilibrium with tropospheric or even urban air. In this sense, groundwater from urban and industrial areas is often contaminated with CFCs, but concentrations are rarely more than a few ppb. The addition of contaminant concentrations of CFCs to groundwater can usually be attributed to anthropogenic point sources such as discharge from septic tanks, leaking sewer lines, leakage from underground storage tanks, discharge or injection of industrial wastes, and recharge from rivers carrying effluent from sewage treatment plants. Thompson and Hayes (1979) found anomalous concentrations of CFC-11 that extended 74 km down gradient along the Balcones fault zone from San Antonio, Texas. The source of the CFC-11 contamination could not be identified, but, because of the narrow shape of the plume, was likely from a point source of approximately 21 kg of CFC-11 spread through approximately 14 km3 of aquifer. The furthest upgradient detection of CFC-11 contamination was in a residential area north-west of the City of San Antonio, Texas, when first detected. Although these waters could not be dated with CFC-11, they demonstrate the potential for large scale tracing of groundwater contamination. Busenberg et al. (1993) used CFCs to map a waste plume at the Idaho National Engineering and Environmental Laboratory in southeast Idaho.
Sewage effluent in rivers has been recognised as a major source of CFC contamination to shallow groundwater (Schultz et al., 1976; Busenberg and Plummer, 1992). Because CFCs were not regulated, CFC contamination to rivers from sewage effluent may have been greater in the 1960s to early 1980s, than today. CFC concentrations in rivers have also decreased since the 1960s/1970s because treatment level (including aeration) has increased. Böhlke et al. (1997) show that halocarbon concentrations in the Danube River rose rapidly in the 1960s and 1970s and then decreased to the present, as recorded in dated groundwater from a shallow aquifer in north-west Hungary. Clark et al. (1995a) found surface water in the Hudson River in the New York City area was contaminated with respect to CFC-11 and CFC-12 by factors of 3 to 5 and 10 to 20, respectively over a 5-month period in 1992. Plummer et al. (1998b) report data on CFC concentrations in river water in south Georgia, south-east USA, sampled at monthly intervals over a 13 month period in 1992-1993. Over this period, CFC-113 was always near equilibrium saturation (0.9 to 1.5 times saturation); CFC-12 was typically near saturation (1.0 to 1.5 times), but with occasional periods of oversaturation of nearly 6 times. Over the 13-month period, CFC-11 was usually oversaturated by 5-20 times, with one sample 137 times saturation. CFC-11, CFC-12, and CFC-113 concentrations in 104 samples of surface water from the Rio Grande and associated drains and laterals near Albuquerque, New Mexico averaged 109± 28, 149± 74, and 112± 19 (± 1s )% of the respective air-water equilibrium values over a 5-month period in 1997 (Plummer et al., 1997).
At ordinary temperatures CFC-113 is liquid (boiling point 47.6°C) with density of 1.565 g cm-3. CFC-113 spills, leakage from underground storage tanks, or release from improper disposal practices pass through soils and sink below the water table. There are several documented cases of gross contamination of groundwater with CFC-113 (Jackson et al., 1992), particularly at some electronics plants in the USA. CFC-11 and CFC-12 are gases at ordinary temperatures (boiling points -23.6 and -29.8°C, respectively), and therefore will normally volatise when released.
Microbial degradation
There is no evidence for aerobic degradation of CFCs in groundwater. Small losses of CFC-11 and CFC-12 were detected in aerobic microcosm experiments with autoclaved sediment and peat (Lovley and Woodward, 1992; Bauer and Yavitt, 1996), but this uptake may have been due to sorption (Bauer and Yavitt, 1996). In contrast, CFC-11, CFC-12 and CFC-113 can all be degraded under anaerobic conditions.
Recent interest in finding natural and synthetic biocatalytic systems that destroy chlorofluorocarbons has contributed research that significantly improves our understanding of microbial processes that degrade CFCs (Sonier et al., 1994; Oremland et al., 1996; Bauer and Yavitt, 1996; Deipser and Stegmann, 1997; Sylvestre et al., 1997). CFCs are electron acceptors, as is nitrate, iron(III), sulphate and CO2 in groundwater. CFCs are degraded primarily by dechlorination reactions that produce hydrochlorofluorocarbons (HCFCs). For example, Sonier et al. (1994) demonstrated that reductive dechlorination of CFC-11 was nearly stoichiometric in the production of fluorodichloromethane (HCFC-21) under sulphate-reducing conditions. In a sulphate-reducing contaminant plume associated with an organic waste disposal site near Ottawa, Ontario, Canada, Lesage et al. (1990) found two dechlorination products (1,2-Dichloro-1,2,2-trifluoroethane (CFC-123a), and chlorotrifluoroethene (CFC-113)), and one defluorination product (1,1,2-trichloro-1,2-difluoroethane (CFC-122a)) of CFC-113.
Deipser and Stegmann (1997) investigated the microbial degradation of CFCs in simulated landfill conditions. Under ‘acidphase' conditions (pH 5.5, 70-90 volume % CO2, 10-30 volume % CH4), created by addition of compost, CFC-11 was degraded to HCFC-21 at an average rate of 0.3 mg/m3material vol./hour, but CFC-12 and CFC-113 were hardly degraded. Under ‘methanephase' conditions (pH 7, 40% CO2, 60% CH4), CFC-11 was again degraded to HCFC-21 with an average rate (11 mg/m3material vol./hour) nearly 37-fold that observed under ‘acidphase' conditions. CFC-12 and CFC-113 also decomposed under ‘methanephase' conditions, both at an average rate of 0.7 mg/m3material vol./hour. Therefore, under methanogenic conditions, Deipser and Stegmann (1997) found CFC-11 degradation to be nearly 16-fold that of CFC-12 and CFC-113. The degradation product of CFC-12, HCFC-22, was apparently stable under the methanic conditions. For analytical reasons, Deipser and Stegmann (1997) were not able to identify the degradation product(s) of CFC-113.
Numerous studies have demonstrated the disappearance of CFCs in natural environments or in laboratory experiments with natural materials (Lovley and Woodward, 1992; Denovan and Strand, 1992; Semprini et al., 1990, 1992; Katz et al., 1995; Cook et al., 1995; Bullister and Lee, 1995; Oster et al., 1996; Shapiro et al., 1997, 1998; Plummer et al., 1998a,b). The results of field observations are generally in agreement with the laboratory observations. Under aerobic conditions, many groundwater studies have demonstrated agreement in apparent ages based on CFC-11, CFC-12 and CFC-113 (Dunkle et al., 1993; Katz et al., 1995; Szabo et al., 1996; Plummer et al., 1998b), which is further evidence of lack of degradation of CFCs under aerobic conditions.
CFC degradation is usually not detected in groundwater until redox conditions reach sulphate reduction. Using biostimulation with acetate, Semprini et al. (1990) observed uptake of CFC-11 and CFC-113 in an anaerobic confined aquifer that began after denitrification was complete. Katz et al. (1995) found CFC-11 apparent ages that were 6-12 years older than those based on CFC-12 in groundwater undergoing sulphate reduction and methanogenesis.
Plummer et al. (1998a, b) mapped concentrations of CFC-11, CFC-12 and CFC-113 in groundwater from the Upper Floridan aquifer in south Georgia, USA over a 650 km2 area impacted by seepage of DOC-rich river water. A wide range of redox conditions, from aerobic to anaerobic sulphate reducing and methanogenic influenced the local groundwater system. CFC-11 and CFC-12 apparent ages were in agreement in aerobic infiltration water, even near the limits of detection of the two compounds. CFC-11 and CFC-113 were nearly absent throughout anaerobic parts of the aquifer that were impacted by seepage of DOC-rich river water, while CFC-12 persisted throughout. The apparent CFC-12 and 3H/3He ages of the river fraction in the mixtures with old groundwater agreed within 5 years or better in 7 of 9 co-dated water samples.
Plummer et al. (1998b) observed degradation of CFC-11 and CFC-113 in sealed ampoules of water from the Withlacoochee river, south-east Georgia which has high dissolved organic carbon content and high concentration of suspended sediment. A large number of ampoules were filled and sealed from a single supply of the river water and stored at room temperature in darkness. Ampoules were analysed at the time of filling and periodically over 2.5 years (Figure 5).
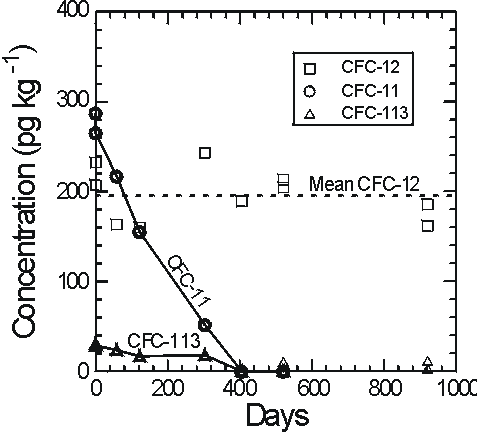
Figure 5 Concentrations of CFC-11, CFC-12 and CFC-113 in sealed ampoules of Withlacoochee River water from south-east Georgia showing degradation of CFC-11 and CFC-113 and apparent stability of CFC-12 (from Plummer et al., 1998b).
CFC-11 and CFC-113 were entirely degraded within 400 days and CFC-12 showed little or no degradation over nearly 1000 days. CFCs were not degraded in ampoules of the river water stored under light at room temperature. Growth of algae was observed in these ampoules which probably sustained aerobic conditions (Plummer et al., 1998b).
Cook et al. (1995) demonstrated nearly complete degradation of CFC-11 and stability of CFC-12 in a sulphate-reducing sand aquifer near Sturgeon Falls, Ontario, Canada. There the groundwater ages based on CFC-12 agreed well with the observed depth of the mid-1960s 3H bomb peak. Oster (1996) reported degradation of CFC-11 and CFC-12 in various (likely methanogenic, but generally unspecified) anoxic environments, with rate of CFC-11 degradation approximately 10-fold that of CFC-12. Under marine, sulphate-reducing conditions in a Norwegian fjord, Shapiro et al. (1997) found rapid degradation of CFC-11, and, within the uncertainties of the measurements, CFC-12 degradation could not have been more than 1/600 that of CFC-11. The half-life for CFC-11 degradation in sulphate-reducing, organic rich seawater was 0.1 ± 0.02 years and considerably smaller than that found by Cook et al. (1995) of 0.9 to 1.7 years.
The presence of sulphate (and nitrate) in anaerobic environments slows the rates of dehalogenation reactions (Mohn and Tiedje, 1992; Sylvestre et al., 1997) by competing with highly halogenated aliphatic compounds as terminal electron acceptors. Inhibition of dehalogenation by sulphate may explain the persistence of CFC-12 in sulphate-reducing environments (as in Cook et al., 1995, Shapiro et al., 1997, and Plummer et al., 1998a,b) where dissolved sulphate is still present, and more rapid degradation of CFC-12 in methanogenic environments (Deipser and Stegmann, 1997; Oster et al., 1996) where sulphate concentrations are usually very low. Parks et al. (1995) reported complete removal of CFC-11 and CFC-12 under methanogenic conditions in the Memphis (sand) aquifer, south-west Tennessee (1.3 mg L-1 CH4, 7.3 mg L-1 SO42-, 5.3 mg L-1 Fe2+). The measured tritium activity of 13.8 TU indicated post-bomb water that would otherwise contain easily measurable concentrations of CFCs. Shapiro et al. (1998) also found significant degradation of CFC-11 and CFC-12 under methanogenic conditions in a buried-valley aquifer near Dayton, Ohio. Fluvial and glacial drift deposits often contain particulate organic carbon and are low in sulphate. Such environments are usually methanogenic, and degradation of CFCs appears to be rapid.
In natural environments the electron donor is usually some form of reduced carbon such as dissolved organic carbon, lignite, or some other form of particulate organic carbon incorporated in the sediment or water column. It is currently not possible to compare absolute rates of degradation from one environment or laboratory reactor to another because of variability in many factors including abundance and diversity of micro-organisms, availability of substrate, organic composition, and presence of possible inhibitors.
Sorption
Like microbial degradation, sorption is another process that can remove CFCs from groundwater, leaving the impression of apparently older age and lower groundwater velocities. Cook et al. (1995) observed uptake of CFC-113 relative to CFC-12 and 3H at the Sturgeon Falls site, Ontario, Canada. The vertical profiles of CFC-12 and tritium could be modelled with a recharge rate of approximately 130 mm yr-1 and were apparently not affected by sorption or degradation. The agreement in transport of CFC-12 and 3H was so strong that, if sorption of CFC-12 were occurring, the solid-liquid partition coefficient, Kd, must be less than 0.03 (retardation factor < 1.15). CFC-113 was retarded relative to CFC-12 and 3H at Sturgeon Falls. Most of the uptake of CFC-113 occurred in the aerobic upper 4 m of the water column, suggesting sorption of CFC-113, rather than microbial degradation (Cook et al., 1995). The CFC-113 profile indicates a Kd value of 0.09-0.14 (retardation factor 1.4-1.7) for CFC-113 in the sand aquifer, which contained, on average, 0.03% organic carbon.
Ciccioli et al. (1980) (see also Cooper, 1981) found no retardation of CFC-11, CFC-12 or CFC-113 relative to chloride in passage through ground Ottawa sand. For ground, washed and dried limestone (Bedford, Indiana), the retardation factors were 1.0, 1.1, and 1.5 for CFC-12, CFC-11, and CFC-113 (Ciccioli et al., 1980), indicating Kd values for these experiments of 0.0, 0.01, and 0.07, respectively (Cook et al., 1995). Brown (1980) measured the retardation of CFC-11 in columns of unground Ottawa sand (very low organic carbon content) and Yolo sandy loam which contained 0.4% organic carbon content. The retardation for CFC-11 was 1.4 (Kd=0.05) for Ottawa sand, and 2.2 (Kd=0.16) for Yolo sandy loam.
Jackson et al. (1992) measured a retardation factor of 12 for CFC-113 in column experiments with CFC-113-spiked uncontaminated Gloucester aquifer water and Gloucester aquifer sediment. The uptake was significantly larger than could be accounted for by the measured organic carbon content of the sediment, leading to the conclusion that other mineral sorbents in the sediment were effective in retarding CFC-113 transport (Jackson et al., 1992).
Russell and Thompson (1983) showed that CFC-11 and CFC-12 sorb to dry soils, and are released when soils are wetted. They proposed this mechanism to explain apparent contamination of CFC-11 and CFC-12 in some shallow groundwater, although there is currently no direct field evidence for this process.
In contrast, Busenberg et al. (1993) and Busenberg and Plummer (unpub. data, 1995) found good agreement between CFC-11, CFC-12 and CFC-113 apparent ages in shallow sand aquifers on the Delmarva Peninsula. Although further study is needed, particularly of sorption potential for CFC-113, sorption does not seem to be important for CFC-11 and CFC-12 in most groundwater environments.
Mixing in the well bore
All groundwater pumped from wells is, to some extent, a mixture. This mixing of water produces ‘mixed ages'. Apparent ages of water sampled from narrow intervals of an aquifer are likely less affected by mixing than those ages interpreted from water samples pumped from large open intervals or from well bores open to multiple fractures or water-bearing zones. In planning for groundwater dating studies, consideration should be given to the range of water ages likely to be intercepted by the wells available for sampling, and whether ages interpreted from such mixtures will be meaningful relative to the goals of the investigation.
Consider the case of N separate and distinct waters mixing in a well bore. If each end-member water sample has a unique and definable age, then we could, in principle, solve for mixing fractions and ages of end-member waters if we measured the concentrations of 2N-1 tracers in the mixture. The problem is easily solved if there is only one water in the ‘mixture'; the apparent age is then defined from the concentration of a single tracer measurement. If two waters mix, we could, in principle, solve the mixing problem with measurement of CFC-11, CFC-12, and CFC-113. In application, the problem becomes rapidly unsolvable as more than 2 waters mix, simply because there are not enough well-established tracers that can be used for dating. Interpretation of mixing problems, even for binary mixtures of young waters, is at best uncertain because, if we could isolate the two end-member waters, the tracer concentrations measured there would likely have some uncertainty in defining end-member age. Nevertheless, the problem could be considered with error simulation techniques and place limits on mixing fractions and ages (Thorstenson et al., 1998; Plummer et al., 1998a, b).
Binary mixtures of young and old waters are more easily interpreted. If the old fraction is CFC-free, and no other processes affect CFC concentrations other than air-water equilibrium, the CFC-11/CFC-12 ratio will define the age of the young fraction if recharged between the late 1940s and about 1977 (Figure 2). Similarly, the CFC-113/CFC-12 ratio will define the age of the young fraction if recharged in the 1980s (Figure 2). (The ratio of SF6/CFC-12 may also prove useful in dating mixtures of young and old water.) Once the age of the young fraction is determined (using tracer ratios), the mixing fractions can be calculated from the measured and expected CFC concentrations.
For binary mixtures of young and old water, the apparent CFC ages of the mixture will almost always be younger than the mean age of the mixture. Figure 6a shows the apparent ages that would be calculated based on CFC-11, CFC-12 and CFC-113, if we assumed a water sample was unmixed, but was actually a mixture of 1940 and 1993 water. The CFC-113 apparent age is biased younger, as much as 5 years, relative to that of CFC-11 and CFC-12, while the CFC-11 and CFC-12 apparent ages appear to be in close agreement. For all CFCs, the apparent age is much younger than the mean age of the water. If 1993 water mixes with water older than 1940, the apparent CFC ages will be unchanged but the mean age will increase from that shown in Figure 6a. The separation between CFC-113 and the other two apparent ages diminishes, however, if the age of the old fraction becomes younger. For mixtures of 1993 water and water recharged in 1975 and more recently, the separation in CFC apparent ages is hardly noticed (Figure 6b), and the apparent ages are similar to the mean groundwater age.
When dating binary mixtures of young and old water, the 3H/3He method (Solomon and Cook, Chapter 13) can be used to determine the age of the young fraction, as the isotope ratio is nearly independent of dilution with old (tritium-free) water (Plummer et al., 1998b). The mixing fraction can then be determined from the CFC concentration.
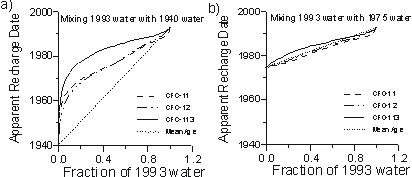
Figure 6 Apparent CFC recharge dates implied if: (a) young water in equilibrium with 1993 air mixes with water recharged in 1940; and (b) young water in equilibrium with 1993 air mixes with water recharged in 1975. Calculations at 10°C and 1 atm. total pressure.
Hydrodynamic dispersion
Because of the smooth and generally increasing nature of the atmospheric mixing ratios, hydrodynamic dispersion is not expected to have a significant effect on CFC concentrations or apparent CFC ages in groundwater. Using a one-dimensional analytical solution to the advection-dispersion equation in steady state flow field (van Genuchten and Alves, 1982), Plummer et al. (1993) simulated the transport and resulting age uncertainties for CFC-11 and CFC-12 for water recharged from 1940 through 1991 (Figure 7). The calculations with CFC-11 and CFC-12 indicate that, if hydrodynamic dispersion were significant, waters recharged since
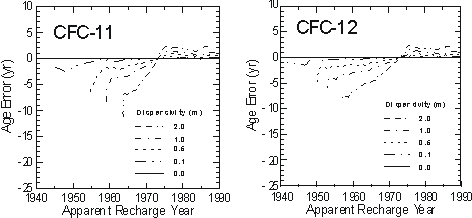
Figure 7 Errors in CFC-11 and CFC-12 apparent ages calculated for one-dimensional flow, with dispersivities of 0 to 2 m (from Plummer et al., 1993).
about 1975 should appear slightly older than the advective age. Water recharged prior to about 1970 would appear to be slightly younger than the advective age. A dispersivity of 0.1 metres has almost no effect on CFC-11 and CFC-12 concentrations over the dating range of the tracers. If dispersivity is 1.0 m, water recharged in 1960 could appear younger relative to the advective age by as much as 5 years for CFC-11 and about 3 years based on CFC-12.
The effect of dispersion on apparent age will be different for tracers that have different shapes to their atmospheric input functions, notably 3H/3He (Solomon and Cook, Chapter 13). In theory, it may be possible to use differences in apparent age derived from different tracers to identify environments where tracer concentrations are strongly affected by hydrodynamic dispersion (Plummer et al., 1993; Ekwurzel et al., 1994), although in practice these small differences may be masked by other processes. A comparison of CFC and 3H/3He apparent ages for waters co-dated from the sand aquifers of the Delmarva Peninsula (Ekwurzel et al., 1994) shows little evidence for significant effects of hydrodynamic dispersion on these tracers. Similarly, Szabo et al. (1996) found good agreement between 3H/3He and CFC ages in a sand aquifer in southern New Jersey, over an age range of nearly 40 years spanning both sides of the 3H bomb peak, indicating that dispersion did not have a significant effect on groundwater ages obtained with these tracers in the study area. Reilly et al. (1994) used CFC dating to refine a groundwater flow model for a shallow sandy aquifer on the Delmarva Peninsula. Comparison of measured tritium with tritium concentrations modelled using a solute transport model corroborated the flow model and demonstrated that effects of hydrodynamic dispersion were negligible in the sand aquifer.
Field Examples
Although much of the research with CFC dating has focused on testing the reliability of the method (see, for example, Dunkle et al., 1993; Ekwurzel et al., 1994; Szabo et al., 1996), there is also a growing literature of successful applications of CFCs in hydrologic investigations. This section summarises some results from selected field studies, and presents brief interpretations of CFC analyses from 10 water samples selected from a wide range of field sites.
Selected applications of CFCs in hydrologic studies
Katz et al. (1995) used CFCs to study leakage from a sinkhole lake in northern Florida and test predictions from a three-dimensional groundwater flow model for transient and steady state flow conditions. To aid in the evaluation of Yucca Mountain, Nevada, as a potential site for a high-level radioactive waste repository, Thorstenson et al. (1998) used CFC measurements of unsaturated-zone air along the mountain crest to show that the travel time of shallow, advecting gas was =5 years.
Plummer et al. (1998a,b) used CFCs to trace recharge of river water to the Upper Floridan aquifer in south-east Georgia derived from seepage through sinkholes in a riverbed. River water seepage was shown to be the sole source of CFC-12 and 3H to the Upper Floridan aquifer, and could be traced some 25 km south-east of the sinkholes in the direction of regional groundwater flow. The fraction of river water in the groundwater mixtures was determined from stable isotope and dissolved chloride data. The apparent ages of the river water fraction, determined from 3H/3He data and from reconstructed CFC-12 concentrations in the river water fraction, were mapped throughout the study area (Figure 8). Velocities of the river water fraction in the saturated karstic aquifer ranged from 0.4 to 8.2 m day-1.
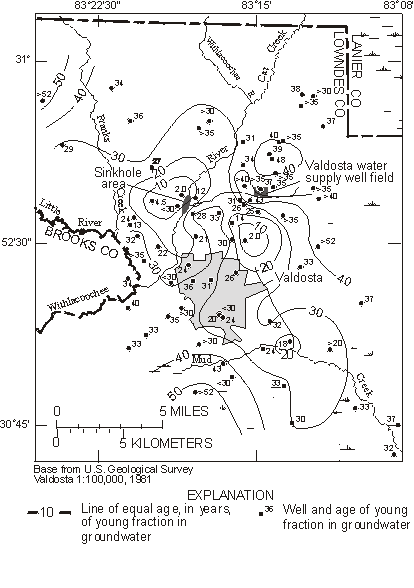
Figure 8 Map showing apparent CFC-12 and 3H/3He ages of the predominant young fraction (river water or regional infiltration water) in groundwater mixtures from the Upper Floridan aquifer near Valdosta, Georgia (from Plummer et al., 1998b).
One of the most important applications of groundwater age data is in providing independent information on recharge rate to aid, for example, in calibration of groundwater flow models (Reilly et al., 1994; Portniaguine and Solomon, 1998). Figure 9 shows a CFC-12 profile measured in a silty sand aquifer near Sturgeon Falls, Ontario, Canada (Cook et al., 1995). Using a two-dimensional flow model, these authors found that the CFC-12 profile was consistent with a recharge flux of 130 mm yr-1 and recharge temperature of 3°C. The location of the tritium peak from the mid-1960s bomb tests in this profile indicated a recharge flux of 135 to 170 mm yr-1.
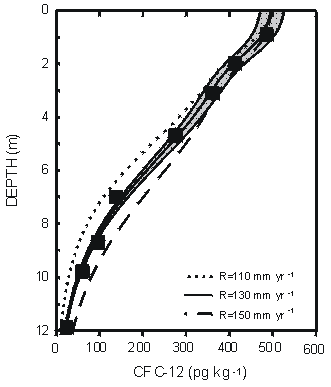
Figure 9 Comparison of CFC-12 concentrations measured in a vertical profile near Sturgeon Falls, Ontario, Canada with a profile simulated using a two-dimensional flow model for assumed recharge rates of 110, 130, and 150 mm yr-1 at a recharge temperature of 3°C. The shaded region represents an uncertainty of ±1 °C in recharge temperature with recharge rate of 130 mm yr-1 (from Cook et al., 1995).
Another important application of CFC dating has been the retrieval of local historical nitrate loading to groundwater from agricultural practices (Dunkle et al., 1993; Böhlke and Denver, 1995; Johnston et al, 1998). For example, Böhlke and Denver (1995) showed that nitrate concentrations in shallow groundwater at an agricultural site along the Atlantic coastal plain of Maryland increased rapidly after the early 1970s, exceeding the drinking water standard of 10 mg L-1 (as N) in recently recharged water. The dated nitrate input to groundwater was used in conjunction with local stream nitrate data and an exponential model of groundwater age to determine the average residence time of shallow groundwater for the area (20 years).
Representative water analyses
We now present some specific examples of water samples for which CFCs and other environmental tracers have been measured, as a means of demonstrating some aspects of interpretation of apparent age from CFC data. Table 3 summarises pertinent data for 10 water samples that demonstrate some of the processes affecting CFC dating discussed previously. The table includes concentrations of dissolved nitrogen and argon used to calculate recharge temperatures and excess air, the concentrations and apparent ages of CFC-11, CFC-12, and CFC-113, and, if available, the measured tritium concentration and 3H/3He age.
Shallow, aerobic infiltration water (Sample 1). Sample 1 (Table 3) is an example of water that is well dated by CFC-11, CFC-12, CFC-113, and 3H/3He. The sample is from a shallow, aerobic sand aquifer on the Delmarva Peninsula of Maryland. There are three 3H/3He dates on samples collected from this monitoring well from April and November,1991 (Ekwurzel et al., 1994), all in the range of 16.0-17.4 years. The CFC-11 and CFC-12 ages measured in 1991 were 19.1 and 19.4, respectively (Ekwurzel et al., 1994). CFC-11, CFC-12, and CFC-113 were measured in July, 1997 at this well giving ages of 16, 17, and 18 years, respectively. Apparently over the 8 years' span in sampling dates, groundwater velocities have been nearly constant.
There are, of course, many more examples of accurately dated waters, but in the following, attention is given to examples exhibiting one or more processes that can affect the reliability of CFC dating.
Sample No. | 1 | 2 | 3 | 4 | 5 | 6 | 7 | 8 | 9 | 10 |
Recharge elev. (m) |
7
|
71
|
47
|
3
|
3
|
77
|
1830
|
1560
|
4
|
561
|
O2 (mg L-1) |
8
|
3.6
|
9
|
0.1
|
.05
|
<0.1
|
5
|
6.7
|
0.02
|
5.9
|
N2 (mg L-1) |
19.9
|
20.0
|
nd
|
nd
|
21.8
|
nd
|
15.5
|
nd
|
31.0
|
21.02
|
Ar (mg L-1) |
0.70
|
0.69
|
nd
|
nd
|
0.68
|
nd
|
0.55
|
nd
|
0.67
|
0.72
|
Recharge temp. (° C) |
11.3
|
16
|
nd
|
9
|
18.2
|
nd
|
13.4
|
nd
|
58
|
7.4
|
Excess air (cm3 L-1) |
2.4
|
3.8
|
nd
|
nd
|
6.4
|
nd
|
1.4
|
nd
|
22.9
|
3.7
|
CFC-11 (pg kg-1) |
488
|
96
|
780
|
63
|
211
|
0
|
181
|
61
|
235
|
299
|
CFC-12 (pg kg-1) |
194
|
3000
|
388
|
2
|
163
|
0
|
111
|
35
|
203
|
131
|
CFC-113 (pg kg-1) |
23
|
6
|
75
|
nd
|
19
|
nd
|
50
|
7
|
30
|
25
|
CFC-11 (yrs) |
16
|
25
|
C
|
28
|
21
|
nd
|
24
|
29
|
21
|
22
|
CFC-12 (yrs) |
17
|
C
|
C
|
47
|
16
|
nd
|
22
|
29
|
14
|
22
|
CFC-113 (yrs) |
18
|
23
|
3
|
nd
|
15
|
nd
|
10
|
25
|
9
|
17
|
Tritium (TU)
|
58.2 - 63.4
|
4
|
10
|
0.0
|
nd
|
13.8
|
0.93
|
0.1
|
13.4
|
11.6
|
3H/3He (yrs)
|
16.0 - 17.4
|
24.9
|
2.5
|
nd
|
nd
|
nd
|
nd
|
nd
|
nd
|
8.3
|
Table 3 Field examples illustrating processes affecting CFC dating of groundwater
(nd = not determined, C = contaminated)
Water contaminated with one or more CFCs (Samples 2-4). Samples 2-4 demonstrate different ways in which groundwater has been contaminated with CFCs (i.e., CFCs have been added in addition to that determined by air-water equilibrium at recharge). Sample 2 is from a domestic well completed in a poorly confined part of the Floridan aquifer near Albany, Georgia. The water is recharged by aerial infiltration into a karstic limestone aquifer, where land use is mostly rural and agricultural. Sample 2 is well dated with CFC-11, CFC-113, and 3H/3He, but is clearly contaminated with CFC-12. The source of the CFC-12 contamination is unknown, but effluent from domestic septic tanks is a possibility. One of the strengths of CFC dating is that there are three compounds capable of defining age, and, depending on land-use practices, contamination with respect to one or two CFCs does not necessarily preclude the possibility of obtaining a reliable age.
It has been suggested that the water shown in Sample 3 represents equilibration with urban air, that is, air somewhat enriched in CFCs relative to that observed at Niwot Ridge, Colorado (Szabo et al., 1996). The water is from a shallow monitoring well in a sand aquifer in southern New Jersey, sampled in April, 1992 in an area where air is affected by two large metropolitan areas, Philadelphia, Pennsylvania and Wilmington, Delaware. At the assumed recharge temperature of 13°C, the measured CFC-11 and CFC-12 concentrations are 110 and 104 pg kg-1 higher than possible for equilibrium with 1992 Niwot Ridge air. At a lower recharge temperature of 11°C, the CFC-11 and CFC-12 concentrations still exceed 1992 air-water equilibrium values by 44 and 77 pg kg-1 respectively. At this site, CFC-113 was apparently not contaminated, indicating an apparent age of 2.8 years (13°C recharge temperature) which compares to 2.5 years based on 3H/3He dating.
Sample 4 is an example of a water sample that was likely contaminated by the sampling equipment. The sample is from the sand aquifer on the Delmarva Peninsula of Maryland, sampled in November, 1991 (Dunkle et al., 1993). The very low tritium content (-0.2 ± 0.3 TU) is consistent with the age of nearly 47 years determined from CFC-12. However, the CFC-11 concentration indicates a recharge date of 1964, and apparent age of 27.6 years. Water recharged in 1964 on the Delmarva Peninsula would have contained more than 100 TU of tritium in 1991 (Dunkle et al., 1993). The sample was collected using a pump that had a moving internal rubber rotor. Dunkle et al. (1993) noted a young bias of CFC-11 apparent ages relative to CFC-12 in many of the older water samples from Delmarva. The addition of small amounts of CFC-11 from sampling equipment would have less effect on younger water with higher CFC-11 concentrations. Subsequent tests comparing water pumped from deep wells with the pump used by Dunkle et al. (1993) and another pump made of all metal parts showed that the earlier-used sample pump introduced CFC-11. Other sampling materials that have been found to contaminate water samples with CFCs include some silicone rubber and plastic tubing used in peristaltic pumps, and plastic impellers and plastic discharge lines used in some pumps.
Microbial degradation of CFCs (Samples 5-6). Samples 5-6 demonstrate effects of microbial degradation on CFCs in groundwater. Sample 5 is from anoxic groundwater on the southern Delmarva Peninsula, Virginia. The water contains little or no dissolved oxygen and a trace of hydrogen sulphide. Apparently, CFC-11 is somewhat degraded, giving an apparent age that is 6 years older than those based on CFC-12 and CFC-113. As a general rule, if dissolved oxygen is less than about 0.5 mg L-1, it is likely that CFC-11 has been at least partially degraded. However, reliable ages can often be obtained from CFC-12 and CFC-113 in such environments.
Sample 6 is from a production well in the Memphis Sand aquifer near the Mississippi River in southwest Tennessee (Parks et al., 1995). The sample contains no detecTable 4issolved oxygen, and large concentrations of ferrous iron (5.3 mg L-1) and methane (1.3 mg L-1). CFC-11 and CFC-12 have been entirely removed, presumably by microbial degradation, leaving the impression of pre-1940s water. However, the tritium measurement of 13.8 TU indicates water with a significant post-bomb fraction. As a general rule, all CFCs can be degraded in low-sulphate, methanic environments.
Groundwater beneath deep unsaturated zones (Samples 7-8). Samples 7 and 8 are from areas in southern Idaho with deep unsaturated zones. Sample 7 is from a monitoring well in the Snake River Plain aquifer, southern Idaho, in an area where groundwater is being recharged by seepage from a nearby stream. The unsaturated zone consists of 16.8 m of alluvial sand that overlies 50.3 m of fractured-basalt and sedimentary-interbed sequence above the water table. Previous work in the area (Busenberg et al., 1993) has shown that diffusive processes control the unsaturated zone air profile in the sand and basalt. Though the sample may be slightly contaminated with CFC-113, the CFC-11 apparent age is about 3 years older than that based on CFC-12, consistent with the diffusive process (Cook and Solomon, 1995).
Sample 8 is from a deep stock well in the Snake River Plain aquifer. The well is 330 m deep, with 308 m of unsaturated zone in fractured basalt and associated sedimentary interbeds. The pump draws from the bottom 25 m of open hole. The well was sampled in 1994 and again in 1995 with consistent observations. The tritium content is only 0.08± 0.01 TU, yet the water contains low, but significant concentrations of CFCs. CFC-11 and CFC-12 concentrations are consistent with equilibration with 1964 air and CFC-113 indicates equilibrium with 1969 air. It is not likely that CFCs could diffuse to such great depths within the past 30 years. However, advective gas flow through fractures driven by barometric pumping and topographic effects likely introduces CFCs to great depths in the unsaturated zone of these fractured basalts. The apparent CFC ages of 25-29 years indicate equilibration with old air, but not the time since recharge. Tritiated infiltration water from the bomb era is apparently still in the unsaturated zone and has not recharged the aquifer. The pre-bomb water at the water table apparently equilibrates with old air and air mixtures that are being transported advectively through the unsaturated zone. If there were no recharge occurring in this semi-arid environment, the presence of CFCs in discharge from the well could indicate that some water is being derived from the top of the water table, perhaps the top 10-20 cm. However, the pump was located at least 10 m below the water table and there was no evidence of excess gases (N2, Ar, He, Ne) in the discharge that would likely be present if water and air from the water table were drawn into the pump. It is more likely that, in this case, the presence of CFCs indicates that some recharge is occurring by infiltration through fractures and sedimentary interbeds in this semi-arid environment.
Gas-stripping and microbial degradation (Sample 9). Products of microbial degradation include carbon dioxide, nitrogen, hydrogen sulphide and methane. As these gases accumulate in groundwater, the sum of the internal partial pressures of all gases can sometimes exceed the hydrostatic pressure at very shallow depths. The result is bubble formation and potential for gas stripping. Gas stripping at the water table will lower the concentrations of all dissolved gases and affect all gas-based dating techniques, including CFCs, 3H/3He, 85Kr, and SF6.
Sample 9 shows potential for gas stripping by nitrogen from denitrification. The sample is from a monitoring well near agricultural fields on the Delmarva Peninsula of Maryland. Under normal recharge conditions, the dissolved nitrogen and argon concentrations should be similar to those of Sample 1. However, Sample 9 contains an excess of approximately 11 mg L-1 of N2, and the argon content is slightly depleted. This is evidence of strong denitrification and possibly a small amount of gas stripping. The total internal pressure of dissolved gases is 1.35 atm, of which dissolved nitrogen totals 1.31 atm. Ekwurzel et al. (1994) noted helium loss from this sample and were unable to date the water with 3H/3He. The CFC-11 apparent age is 7 years older than that based on CFC-12 and may indicate degradation of CFC-11. The CFC-12 and CFC-113 ages of 9 to 14 years are not inconsistent with the tritium data, but, if affected by gas stripping, represent a maximum age.
Groundwater mixtures (Sample 10). Sample 10, from a water supply well completed in fractured crystalline rock in Shenandoah National Park, Virginia, shows the effect of mixing of young and old groundwater. Drilling records show only two water-bearing zones, each about a metre wide, at 183 and 191 m depth and more than 120 m below the water level in the well. The well has only 15.8 m of casing. The CFC and 3H/3He data indicate that the well likely produces a mixture of young and old water. The mixing may occur in the well bore, but more likely within the fracture network in the vicinity of the well induced by stress from pumping. If the discharge from the well is a mixture of young and older (pre-bomb) water, the 3H/3He age of 8.3 years applies to the young fraction. If the young fraction was recharged in 1988 (based on the sampling date of 1996.3), it would have contained 759, 310, and 80.6 pg kg-1 of CFC-11, CFC-12, and CFC-113 respectively, at the average local recharge temperature of 8 °C and 561 m elevation. The measured concentrations of CFC-11, CFC-12, and CFC-113 indicate that, if a binary mixture of old and 1988 water, the mixture contains between 30 and 42% of 1988 water.
Concluding Remarks
In cases such as Sample 1, above, where all three CFCs indicated very similar ages, considerable confidence can be placed in the interpreted apparent age. In general, CFC dating is most likely to be successful in rural settings, with shallow water tables, where the groundwater is aerobic, and not impacted by local contaminant sources such as septic tanks or industrial applications. In the examples above, assignment of apparent age based on CFCs was, however, not always straightforward. As we enter more complex hydrogeochemical environments, we will need to rely increasingly on additional data in interpreting apparent ages from CFCs. Measurements of concentrations of dissolved gases, such as dissolved oxygen, to determine if there is potential for microbial degradation, can be extremely useful. Noble gas measurements are useful in defining recharge temperature and excess air. Measurements of dissolved nitrogen and argon can also be used to determine recharge temperature, excess air, and recognise environments undergoing denitrification. Measurements of dissolved methane are useful in recognising environments in which all three CFCs can be degraded. Tritium measurements have proven particularly useful in helping to validate CFC apparent ages. A useful test is to reconstruct initial tritium concentrations in precipitation using the CFC apparent age and measured tritium content for comparison with expected historical tritium-precipitation data (e.g., Busenberg and Plummer, 1992; Dunkle et al., 1993; Ekwurzel et al., 1994; Plummer et al., 1998b). In cases of mixing of young water with old water (old water on the 1000-10 000 year time scale), 14C can be useful in estimating mixing fractions. In CFC-contaminated environments or methanogenic environments, noble gas dating techniques such as those based on 3H/3He and 85Kr measurements may provide reliable dating information. Because of their low detection limit and low sensitivity to hydrodynamic dispersion, CFCs may be more reliable than 3H/3He and 85Kr dating for waters recharged in the late 1940s and 1950s. All dating techniques have limitations, and, therefore, greater confidence in apparent age will be realised as multiple dating techniques are applied to the same sample.
References
AFEAS (1997) Production, sales and atmospheric release of fluorocarbons through 1995. Alternative Fluorocarbons Environmental Acceptability Study Program Office, The West Tower - Suite 400, 1333 H Street NW, Washington, DC 20005, U.S.A.
Bauer M.R. and Yavitt J.B. (1996) Processes and mechanisms controlling consumption of CFC-11 and CFC-12 by peat from a conifer-swamp and black spruce-tamarack bog in New York State. Chemosphere 32(4), 759-768.
Böhlke J.K. and Denver J.M. (1995) Combined use of groundwater dating, chemical, and isotopic analyses to resolve the history and fate of nitrate contamination in two agricultural watersheds, Atlantic coastal plain, Maryland. Water Resour. Res. 31(9), 2319-2339. J., Deseo E. and Stute M. (1997) Groundwater record of halocarbon trans
Böhlke J.K., Revesz K., Busenberg E., Deak port by the Danube River. Environ. Sci. Technol., 31(11), 3293-3299.
Bönisch G., Blindheim J., Bullister J.L., Schlosser P. and Wallace D.W.R. (1997) Long-term trends of temperature, salinity, density, and transient tracers in the central Greenland Sea. J. Geophys. Res. - Oceans 102(C8), 18553-18571.
Brown J.D. (1980) Evolution of Fluorocarbon Compounds as Ground Water Tracers-Soil Column Studies. M.S. Thesis. Department of Hydrology Water Resources, University Arizona, Tuscon, 97pp.
Bu X. and Warner M.J. (1995) Solubility of chlorofluorocarbon 113 in water and seawater. Deep-Sea Res. 42(7), 1151-1161.
Bullister J.L. (1984) Atmospheric chlorofluomethanes as tracers of ocean circulation and mixing: Studies in the Greenland and Norwegian seas. Ph.D. Dissertation, University of Califorina, San Diego, La Jolla, 172pp.
Bullister J.L. (1989) Chlorofluorocarbons as time-dependent tracers in the ocean. Oceanography 2, 12-17.
Bullister J.L. and Lee B.S. (1995) Chlorofluorocarbon-11 removal in anoxic marine waters. Geophys. Res. Lett. 22(14), 1893-1896.
Bullister J. L. and Weiss R.F. (1988) Determination of CCl3F and CClF2 in seawater and air. Deep-Sea Res. 35(5), 839-854.
Busenberg E. and Plummer L.N. (1991) Chlorofluorocarbons (CCl3F and CCl2F2): Use as an age dating tool and hydrologic tracer in shallow ground-water systems. In Proceedings, U.S. Geological Survey Toxic Substances Hydrology Program, Technical Meeting. Monterey, California, March 11-15, 1991, eds. G. L. Mallard and D. A. Aronson, U.S. Geological Survey Water-Resources Investigations Report 91-4034, pp. 542-547.
Busenberg E. and Plummer L.N. (1992) Use of chlorofluorocarbons (CCl3F and CCl2F2) as hydrologic tracers and age-dating tools: The alluvium and terrace system of Central Oklahoma. Water Resour. Res. 28(9), 2257-2283.
Busenberg E. and Plummer L.N. (1993) Use of trichlorofluorocarbon-113 (CFC-113) as a hydrologic tracer and age-dating tool for young groundwater. Geological Society of America, 1993 Annual Meeting, Abstracts with Programs, 25(6), A-365.
Busenberg E. and Plummer L.N. (1997) Use of sulfur hexafluoride as a dating tool and as a tracer of igneous and volcanic fluids in ground water. Geological Society of America, 1997 Annual Meeting, Abstracts with Programs 29(6), A-78.
Busenberg E., Weeks E.P., Plummer L.N. and Bartholemay R.C. (1993) Age dating ground water by use of chlorofluorocarbons (CCl3F and CCl2F2), and distribution of chlorofluorocarbons in the unsaturated zone, Snake River Plain aquifer, Idaho National Engineering Laboratory, Idaho. U.S. Geological Survey Water-Resources Investigations 93-4054, 47.
Ciccioli P., Cooper W.T., Hammer P.M. and Hayes J.M. (1980) Organic solute-mineral surface interactions: A new method for the determination of groundwater velocities. Water Resour. Res. 16(1), 217-223.
Clark J.F., Smethie W.M., Jr. and Simpson H.J. (1995a) Chlorofluorocarbons in the Hudson estuary during summer months. Water Resour. Res. 31(10), 2553-2560.
Clark J.F., Simpson H.J., Bopp R.F. and Deck B.L. (1995b) Dissolved oxygen in lower Hudson Estuary: 1978-93. J. Environ. Engineer. 121, 760-763.
Cook P.G. and Solomon D.K. (1995) The transport of atmospheric trace gases to the water table: Implications for groundwater dating with chlorofluorocarbons and Krypton-85. Water Resour. Res. 31(2), 263-270.
Cook P.G. and Solomon D.K. (1997) Recent advances in dating young groundwater: chlorofluorocarbons, 3H/3He, and 85Kr. J. Hydrol. 191, 245-265.
Cook P.G., Solomon D.K., Plummer L.N., Busenberg E. and Schiff S.L. (1995) Chlorofluorocarbons as tracers of groundwater transport processes in a shallow, silty sand aquifer. Water Resour. Res. 31(3), 425-434.
Cook P.G., Solomon D.K., Sanford W.E., Busenberg E., Plummer L.N. and Poreda R.J. (1996) Inferring shallow groundwater flow in saprolite and fractured rock using environmental tracers. Water Resour. Res. 32(6), 1501-1509.
Cooper W.T. (1981) Interactions Between Organic Solutes and Mineral Surfaces and their Significance in Hydrogeology. Ph.D. Dissertation, Indiana University, Bloomington, Indiana, 234pp.
Cunnold D.M., Weiss R.F., Prinn R.G., Hartley D., Simmonds P.G., Fraser P.J., Miller B., Alyea F.N. and Porter L. (1997) GAGE/AGAGE measurements indicating reductions in global emissions of CCl3F and CCl2F2 in 1992-1994. J. Geophys. Res.-Atmos. 102(D1), 1259-1269.
Deipser A. and Stegmann R. (1997) Biological degradation of VCCs and CFCs under simulated anaerobic landfill conditions in laboratory test digesters. Environ. Sci. Pollut. Res. Int. 4(4), 209-216.
Denovan D.A. and Strand S.E. (1992) Biodegradation of chlorofluorocarbons in anaerobic environments. Chemosphere 24, 935-940.
Dunkle S.A., Plummer L.N., Busenberg E., Phillips P.J., Denver J.M., Hamilton P.A., Michel R.L. and Coplen T.B. (1993) Chlorofluorocarbons (CCl3F and CCl2F2) as dating tools and hydrologic tracers in shallow ground water of the Delmarva Peninsula, Atlantic coastal plain, United States. Water Resour. Res. 29(12), 3837-3860.
Ekwurzel B., Schlosser P., Smethie W.M., Jr., Plummer L.N., Busenberg E., Michel R.L., Weppernig, R. and Stute M. (1994) Dating of shallow groundwater: Comparison of the transient tracers 3H/3He, chlorofluorocarbons and 85Kr. Water Resour. Res. 30(6), 1693-1708.
Elkins J.W., Thompson T.M., Swanson T.H., Butler J.H., Hall B.D., Cummings S.O., Fisher D.A. and Raffo A.G. (1993) Decrease in the growth rates of atmospheric chlorofluorocarbons 11 and 12. Nature 364, 780-783.
Elkins J.W. (Editor), Butler J.H., Thompson T.M., Montzka S.A., Myers R.C., Lobert J.M., Yvon S.A., Wamsley P.R., Moore F.L., Gilligan J.M., Hurst D.F., Clarke A.D., Swanson T.H., Volk C.M., Lock L.T., Geller L.S., Dutton G.S., Dunn R.M., Dicorleto M.F., Baring T.J. and Hayden A.H. (1996) Nitrous oxide and halocompounds. In Climate Monitoring and Diagnositcs Laboratory No. 23, Summary Report 1994-1995. eds. D.J. Hofmann, J.T. Peterson and R.M. Rosson, U.S. Department of Commerce, NOAA Environmental Research Laboratories, pp. 84-111.
Epler N.A. (1990) Chlorofluoromethanes as tracers of recent ground water on Long Island, New York. NWWA Annual Meeting, Anaheim, CA, Sept. 25-26, 1990, p.8.
Fisher D.A. and Midgley P.M. (1993) Production and release to the atmosphere of CFCs 113, 114 and 115. Atmos. Environ. 27A, 271-276.
Fraser P., Cunold D., Alyea F., Weiss R., Prinn R., Simmonds P., Miller B. and Langenfelds R. (1996) Lifetime and emission estimates of 1, 1, 2-trichlorotrifluorethane (CFC-113) from daily global background observations June 1982-June 1994. J. Geophys. Res. - Atmos. 101(D7), 12585-12599
Gamlen P.H., Lane B.C., Midgley P.M. and Steed J.M. (1986) The production and release to the atmosphere of CCl3F and CCl2F2 (chlorofluorocarbons CFC 11 and CFC 12). Atmos. Environ. 20, 1077-1085.
Gee G. W. and Hillel D. (1988) Ground-water recharge in arid regions: Review and critique of estimation methods. Hydrol. Proc. 2, 255-266.
Geller L.S., Elkins J.W., Lobert J.M., Clarke A.D., Hurst D.F., Butler J.H. and Myers R.C. (1997) Tropospheric SF6: Observed latitudinal distribution and trends, derived emissions and interhemispheric exchange time. Geophys. Res. Lett. 44(6), 675-678.
Hayes J.M. and Thompson G.M. (1977) Trichlorofluoromethane in groundwater - A possible indicator of groundwater age. Water Resources Research Center, Technical Report 90, Purdue University, NTIS Report PB 265 170, 25p.
Ho D.T., Schlosser P., Smethie W.M., Jr. and Simpson H.J. (1998) Variability in atmospheric chlorofluorocarbons (CCl3F and CCl2F2) near a large urban area: Implications for groundwater dating. Environ. Sci. Technol. 32(16), 2377-2382.
Hofer M. and Imboden D.M. (1998) Simultaneous determination of CFC-11, CFC-12, N2 and Ar in water. Anal. Chem. 70, 724-729.
Hurst D.F., Bakwin P.S., Myers R.C. and Elkins J.W. (1997) Behavior of trace gas mixing ratios on a very tall tower in North Carolina. J. Geophys. Res. - Atmos. 102(D7), 8825-8835.
Jackson R.E., Lesage S. and Priddle M.W. (1992) Estimating the fate and mobility of CFC-113 in groundwater: Results from the Gloucester landfill project. In Groundwater Contamination and Analysis at Hazardous Waste Sites, eds. S. Lesage and R.E. Jackson, pp. 511-526, Marcel Dekker, New York, N.Y.
Jean-Baptiste P., Messias M.J., Alba C., Charlou J.L. and Bougault H. (1994) A simple copper-tube sampler for collecting and storing seawater for post-cruise CFC measurements. Deep-Sea Res. 41(9), 1361-1372.
Johnston C.T., Cook P.G., Frape S.K., Plummer L.N., Busenberg E. and Blackport R.J. (1998) Ground water age and nitrate distribution within a glacial aquifer beneath a thick unsaturated zone. Ground Water 36(1), 171-180.
Kanakidou M., Dentener F.J. and Crutzen P.J. (1995) A global three-dimensional study of the fate of HCFCs and HFC-134a in the troposphere. J. Geophys. Res. - Atmos. 100(D9), 18781-18801.
Katz B.G., Lee T.M., Plummer L.N. and Busenberg E. (1995) Chemical evolution of groundwater near a sinkhole lake, northern Florida. 1. Flow patterns, age of groundwater, and influence of lakewater leakage. Water Resour. Res. 31(6), 1549-1564.
Ko M.W., Sze N.D., Wang W.-C., Shia G., Goldman A., Murcray F.J., Murcray D.G. and Rindland C.P. (1993) Atmospheric sulfur hexafluoride: Sources, sinks and greenhouse warming. J. Geophys. Res.- Atmos. 98(D6), 10499-10507.
Law C.S., Watson A.J. and Liddicoat M.I. (1994) Automated vacuum analysis of sulfur hexafluoride in seawater: derivation of the atmospheric trend (1979-1993) and potential as a transient tracer. Mar. Chem. 48, 57-69.
Lesage S., Jackson R.E., Priddle M.W. and Riemann P.G. (1990) Occurrence and fate of organic solvent residues in anoxic groundwater at the Gloucester Landfill, Canada. Environ. Sci. Technol. 24, 559-566.
Levin I. and Hesshaimer V. (1996) Refining of atmospheric transport model entries by the globally observed passive tracer distributions of 85krypton and sulfur hexafluoride (SF6). J. Geophys. Res - Atmos. 101(D11), 16745-16755.
List R.J. (1949) Smithsonian Meteorological Tables. Sixth ed., p.527, Smithsonian Institution Press, Washington, D.C.
Lobert J.M., Butler J.H., Baring T.J., Montzka S.M., Myers R.C. and Elkins J.W. (1995) OAXTC 92: Ocean/Atmosphere Exchange of Trace Compounds 1992: Oceanic measurements of HCFC-22, CFC-11, CFC-12, CFC-113, CH3CCl3, CCl4, and N2O in the marine air and surface waters of the west Pacific Ocean (August 3-October 21, 1992). NOAA Technical Memorandum, ERL CMDL-9, p.43.
Lovelock J.E. (1971) Atmospheric fluorine compounds as indicators of air movements. Nature 230, 379.
Lovley D.R. and Woodward J.C. (1992) Consumption of Freon CFC-11 and CFC-12 by anaerobic sediments and soils. Environ. Sci. Technol. 26, 925-929.
Maiss M., Steele L.P., Francey R.J., Fraser P.J., Langenfelds R.L., Trivett N.B.A. and Levin I. (1996) Sulfur hexafluoride - A powerful new atmospheric tracer. Atmos. Environ. 30(10-11), 1621-1629.
Massmann J. and Farrier D.F. (1992) Effects of atmospheric pressures on gas transport in the vadose zone. Water Resour. Res. 28(3), 777-791.
McCarthy R.L., Bower F.A. and Jesson J.P. (1977) The fluorocarbon-ozone theory-1. Production and release - World production and release of CCl3F and CCl2F2 (Fluorocarbons 11 and 12) through 1975. Atmos. Environ. 11, 491-497.
McCulloch A., Midgley P.M. and Fisher D.A. (1994) Distribution of emissions of chlorofluorocarbons (CFCs) 11, 12, 113, 114, and 115 among reporting and non-reporting countries in 1986. Atmos. Environ. 28, 2567-2582.
Midgley P.M. and Fisher D.A. (1993) Production and release to the atmosphere of chlorodifluoromethane (HCFC-22). Atmos. Environ. 27A, 2215-2223.
Mohn W.W. and Tiedje J.M. (1992) Microbial reductive dehalogenation. Microbiology Review 56, 482-507.
Montzka S.A., Myers R.C., Butler J.H., Elkins J.W., Lock L.T., Clarke A.D. and Goldstein A.H. (1996) Observations of HFC-134a in the remote troposphere. Geophys. Res. Lett. 23(2), 169-172.
Morris R.A., Miller T.M., Viggiano A.A., Paulson F.J., Solomon S. and Reid G. (1995) Effects of electron and ion reactions on atmospheric lifetimes of fully fluorinated compounds. J. Geophys. Res. - Atmos. 100(D1), 1287-1294.
Mroczek E.K. (1997) Henry's law constants and distribution coefficients of sulfur hexafluoride in water from 25 ° C to 230 ° C. J. Chem. Eng. Data 42(1), 116-119.
Nilson R.H., Peterson E.W., Lie K.H., Burkhard N.R. and Hearst J.R. (1991) Atmospheric pumping: A mechanism causing vertical transport of contaminated gases through fractured permeable media. J. Geophys. Res. - Solid Earth 96(B13), 21933-21948.
Oram D.E., Sturges W.T., Penkett S.A., McCulloch A. and Fraser P.J. (1998) Growth of fluoroform (CHF3, HFC-23) in the background atmosphere. Geophys. Res. Lett. 25(1), 35-38.
Oremland R.S., Lonergan D.J., Culbertson C.W. and Lovley D.R. (1996) Microbial degradation of hydrochlorofluorocarbons (CHCl2F and CHCl2CF3) in soils and sediments. Appl. Environ. Microbiol. 62(5), 1818-1821.
Oster H., Sonntag C. and Munnich K.O. (1996) Groundwater age dating with chlorofluorocarbons. Water Resour. Res. 32(10), 2989-3001.
Parks W.S., Mirecki J.E. and Kingsbury J.A. (1995) Hydrogeology, ground-water quality, and source of ground water causing water-quality changes in the Davis well field at Memphis, Tennessee. U.S. Geological Survey Water-Resources Investigation Report. 94-4212, 58p.
Plummer L.N., McConnell J.B., Busenberg E., Drenkard S., Schlosser P. and Michel R.L. (1998a) Flow of river water into a karstic limestone aquifer—1. Tracing the young fraction in groundwater mixtures in the Upper Floridan aquifer near Valdosta, Georgia. Appl. Geochem. 13(8), 995-1015..
Plummer L.N., Busenberg E., Drenkard S., Schlosser P., McConnell J.B., Michel R.L., Ekwurzel B. and Weppernig R. (1998b) Flow of river water into a karstic limestone aquifer—2. Dating the young fraction in groundwater mixtures in the Upper Floridan aquifer near Valdosta, Georgia. Appl. Geochem. 13(8), 1017-1043
Plummer L.N., Busenberg E., Sanford W. E., Bexfield L.M. and Anderholm S.K. (1997) Tracing and dating young ground water in the Middle Rio Grande Basin, Albuquerque, New Mexico. Geological Society of America, 1997 Annual Meeting, GSA Abstracts with Programs 29(6), A-135-136.
Plummer L.N., Michel R.L., Thurman E.M. and Glynn P.D. (1993) Environmental tracers for age-dating young ground water. In Regional Ground-Water Quality, ed. W.M. Alley, pp. 255-294, Van Nostrand Reinhold, New York, N.Y.
Portniaguine O. and Solomon D.K. (1998) Parameter estimation using groundwater age and head data, Cape Cod, Massachusetts. Water Resour. Res. 34(4), 637-645.
Prather M.J. (1995) Atmospheric lifetimes of HCFCs and HFCs: Current estimates and uncertainties. Proceedings, NASA/NOAA/AFEAS Workshop on the Atmospheric Degradation of HCFCs and HFCs, November 17-19, 1993, Boulder, Colorado, pp. 1.1-1.19.
Prinn R.G., Weiss R.F., Miller B.R., Huang J., Alyea F.N., Cunnold D.M., Fraser P.J., Hartley D.E. and Simmonds P.G. (1995) Atmospheric trends and lifetime of CH3CCl3 and global OH concentrations. Science 269, 187-192.
Randall J.H. and Schultz T.R. (1976) Chlorofluorocarbons as hydrologic tracers: A new technology. Hydrology Water Resources Arizona Southwest 6, 189-195.
Randall J.H., Schultz T.R. and Davis S.N. (1977) Suitability of fluorocarbons as tracers in ground water resources evaluation. Technical report to Office of Water Research and Technology, U.S. Department of the Interior, NTIS PB 277 488, 37pp.
Ravishankara R.A., Solomon S., Turnipseed A.A. and Warren R.F. (1993) Atmospheric lifetimes of long-lived species. Science 259, 194-199.
Reilly T.E., Plummer L.N., Phillips P.J. and Busenberg E. (1994) The use of simulation and multiple environmental tracers to quantify groundwater flow in a shallow aquifer. Water Resour. Res. 30(2), 421-433.
Rhein M. (1991) Ventilation rates of the Greenland and Norwegian Seas derived from distributions of the chlorofluoromethanes F11 and F12. Deep-Sea Res. 38, 485-503.
Russell A.D. and Thompson G.M. (1983) Mechanism leading to enrichment of the atmospheric fluorocarbons CCl3F and CCl2F2 in groundwater. Water Resour. Res. 19(1), 57-60.
Schlatter J.W., Wuest A. and Imboden D.M. (1997) Hypolimnetic density currents traced by sulfur hexafluoride (SF6). Aquat. Sci. 59, 225-242.
Schlosser P., Stute M., Sonntag C. and Munnich K.O. (1989) Tritiogenic 3He in shallow groundwater. Earth Planet. Sci. Lett. 94, 245-256.
Schultz T.R. (1979) Trichlorofluoromethane as a ground-water tracer for finite-state models. Ph.D. Dissertation, University of Arizona.
Schultz T.R., Randall J.H., Wilson L.G. and Davis S.N. (1976) Tracing sewage effluent recharge- Tucson, Arizona. Ground Water 14, 463-470.
Semprini L., Hopkins G.D., McCarty P.L. and Roberts P.V. (1992) In-situ transformation of carbontetrachloride and other compounds resulting from biostimulation under anoxic conditions. Environ. Sci. Technol. 26, 2454-2461.
Semprini L., Hopkins G.D., Roberts P.V. and McCarty P.L. (1990) In-situ biotransformation of carbon tetrachloride, 1,1,1,-trichloroethane, Freon-11, and Freon-12 under anoxic conditions. Eos, Trans. Am. Geophys. Union 71, 1324.
Severinghaus J.P., Keeling R.F., Miller B.R., Weiss R.F., Deck B. and Broecker W.S. (1997) Feasibility of using sand dunes as archives of old air. J. Geophys. Res. - Atmos. 102(D14), 16783-16792.
Shapiro S.D., Schlosser P., Smethie W.M., Jr. and Stute M. (1997) The use of 3H and tritiogenic 3He to determine CFC degradation and vertical mixing rates in Framvaren Fjord, Norway. Mar. Chem. 59, 141-157.
Shapiro S.D., Rowe G., Schlosser P., Ludin A. and Stute M. (1998) Tritium-helium 3 dating under complex conditions in hydraulically stressed areas of a buried-valley aquifer. Water Resour. Res. 34(5), 1165-1180.
Smethie W.M. (1993) Tracing the thermohaline circulation in the western North Atlantic using chlorofluorocarbons. Prog.Oceanogr. 31, 51-99.
Solomon D.K., Schiff S.L., Poreda R.J. and Clark W.B. (1993) A validation of the 3H/3He method for determining groundwater recharge. Water Resour. Res. 29(9), 2951-2962.
Sonier D.N., Duran N.L. and Smith G.B. (1994) Dechlorination of trichlorofluoromethane (CFC-11) by sulfate-reducing bacteria from an aquifer contaminated with halogenated aliphatic compounds. Appl. Environ. Microbiol. 60, 4567-4572.
Sylvestre M., Bertrand J.L. and Viel G. (1997) Feasibility study for the potential use of biocatalytic systems to destroy chlorofluorocarbons (CFCs). Crit. Rev. Environ. Sci. Technol. 27(2), 87-111.
Szabo Z., Rice D.E., Plummer L.N., Busenberg E., Drenkard S. and Schlosser P. (1996) Age-dating of shallow groundwater with chlorofluorocarbons, tritium/helium 3, and flow path analysis, southern New Jersey coastal plain. Water Resour. Res. 32(4), 1023-1038.
Thompson G.M. (1976) Trichloromethane: A New Hydrologic Tool for Tracing and Dating Groundwater. Ph.D. Dissertation, Department of Geology, Indiana University, Bloomington, Indiana. 93pp.
Thompson G.M. and Hayes J.M. (1979) Trichlorofluoromethane in groundwater: A possible tracer and indicator of groundwater age. Water Resour. Res. 15(3), 546-554.
Thompson G.M., Hayes J.M. and Davis S.N. (1974) Fluorocarbon tracers in hydrology. Geophys. Res. Lett. 1, 177-180.
Thompson T.M., Komhyr W.D. and Dutton E.G. (1985) Chlorofluorocarbon-11, -12 and nitrous oxide measurements at the NOAA/GMCC baseline stations (16 September 1973 to 31 December 1979). NOAA Technical Report ERL428-ARL 8, p.124 .
Thorstenson, D.C., Weeks E.P., Haas H., Busenberg E., Plummer L.N. and Peters C.A. (1998) Chemistry of unsaturated zone gases sampled in open boreholes at the crest of Yucca Mountain, Nevada: Data and basic concepts of chemical and physical processes in the mountain. Water Resour. Res. 34(6), 1507-1529.
Tominaga T. (1992) Chlorofluorocarbons in the atmosphere: Trends and vertical profiles. Pure Appl. Chem. 64, 529-536.
van Genuchten M.Th. and Alves W.J. (1982) Analytical solutions of the one-dimensional convective-dispersion solute transport equation. U.S. Department Agriculture Technical Bulletin 1661, 151p.
Volk C.M., Elkins J.W., Fahey D.W., Dutton G.S., Gilligan J.M., Loewenstein M., Podolske J.R., Chan K.R. and Gunson M.R. (1997) Evaluation of source gas lifetimes from stratospheric observations. J. Geophys. Res. - Atmos. 102(D21), 25543-25564.
Wallace D.W.R., Beining P. and Putzka A. (1994) Carbon tetrachloride and chlorofluorocarbons in the South Atlantic Ocean, 19°S. J. Geophys. Res. - Oceans 99(C4), 7803-7819.
Wang J.-L., Chang, C.-J. and Lin, Y.-H. (1998) Concentration distributions of anthropogenic halocarbons over a metropolitan area. Chemosphere 36(10), 2391-2400.
Wanninkhof R., Ledwell J. R. and Watson A.J. (1991) Analysis of sulfur hexafluoride in seawater. J. Geophys. Res. - Oceans 96(C5), 8733-8740.
Warner M.J. and Weiss R.F. (1985) Solubilities of chlorofluorocarbons 11 and 12 in water and seawater. Deep-Sea Res. 32, 1485-1497.
Warner M.J., Bullister J.L., Wisegarver D.P., Gammon R.H. and Weiss R.F. (1996) Basin-wide distributions of chlorofluorocarbons CFC-11 and CFC-12 in the North Pacific: 1985-1989. J. Geophys. Res. - Oceans 101(C9), 20525-20542.
Weeks E.P., Earp D.E. and Thompson G.M. (1982) Use of atmospheric fluorocarbons F-11 and F-12 to determine the diffusion parameters of the unsaturated zone in the southern high plains of Texas. Water Resour. Res. 15, 1365-1378.
Weiss R.F. and Price B.A. (1980) Nitrous oxide solubility in water and seawater. Mar. Chem. 8, 347-359.
Wilkowske C.D. and Solomon D.K. (1997) Evaluation of a simple copper tube sampler for dating ground water with chlorofluorocarbons (CFCs). Geological Society of America, 1997 Annual Meeting, GSA Abstracts with Programs, 29(6), A-77.
Wilson G.B. and McNeill G.W. (1997) Noble gas recharge temperatures and excess air component. Appl. Geochem. 12, 747-762.
Wilson R.D. and Mackay D.M. (1995) Direct detection of residual nonaqueous phase liquid in the saturated zone using SF6 as a partitioning tracer. Environ. Sci. Technol. 29(5), 1255-1258.
Wisegarver D.P. and Gammon R.H. (1988) A new transient tracer: Measured vertical distribution of CCl2FCClF2 (F-113) in the North Atlantic subarctic gyre. Geophys. Res. Lett. 15, 188-191.